Introduction
The brewing process generates different wastes and by-products that can be valorized by implementing biorefinery techniques (Olajire, 2020; Mussatto, et al., 2013). In a brewery, malted barley is milled and mixed with water in a mash tun where temperature slowly increases from 37 to 78°C to promote enzymatic hydrolysis of malt constituents. This enzymatic conversion stage (mashing) produces a sweet liquid known as wort which later is boiled in presence of hops and cooled to be used as a fermentation medium to produce beer. The insoluble, undegraded malted barley grain forms a bed within the mash tun and the sweet wort is filtered through it. The residual solid fraction from mashing is known as brewer's spent grain (BSG) (Mussatto, et al., 2006). Approximately 20 kg of wet BSG are generated per 100 L of brewed beer (Steiner, et al., 2015). The Colombian beer production in 2019 was about 23.835.228 hL (DANE, 2019), which means that 476.705 t of wet BSG were generated.
BSG is the most abundant by-product in the beer industry representing approximately 85% (w/w) of the total wastes and by-products from the beer-brewing process (Lynch, et al., 2016). It is mainly composed of lignin, cellulose, hemicellulose, and proteins (Steiner, et al., 2015; Wang, et al., 2015). Due to its composition, high availability, and low cost, BSG is recognized by different authors as a valuable renewable resource for industry (Paz, et al., 2019; Puligundla & Mok, 2021; Corchado-lopo, et al., 2021) with potential applications in the pharmaceutical, food, cosmetics, personal care, polishes, and coatings industries, as well as in other sectors including the production of liquid biofuels ( Shen, et al., 2019; Jackowski, et al., 2020).
BSG valorization through the production of chemical compounds requires hydrolysis to obtain cellulose and hemicellulose, raw materials used for the production of reducing sugars (Mussatto, 2014). Although BSG hydrolysis results from the action of acids, alkalis, or enzymes (Rafiqul, et al., 2014; Jackowski, et al., 2020), the use of the latter has several advantages compared to chemicals for food and feed applications, besides a more precise control of the process, the nature of the components yielded, and the production of fractions that retain bioactivity. Additionally, as enzymatic processes do not generate potentially toxic side streams, they are considered more environmentally friendly (Lynch, et al., 2016) and inherently safe.
A promising chemical product that can be obtained from BSG by implementing biorefinery techniques is xylitol, a functional sweetener with important applications in the food and pharmaceutical industries (Swart, et al., 2021). Xylitol production requires xylose, which is the result of the complex mixture of cellulose, hemicellulose, and lignin from BSG (Carvalheiro, et al., 2006; Mussatto & Roberto, 2008). Partial BSG degradation to release xylose and other sugars has been reported in previous works with enzyme extracts from Bacillus subtilis (Moteshafi, et al., 2016) and Fusarium oxysporum (Puligundla & Mok, 2021). To carry out complete and efficient BSG enzymatic hydrolysis, the action of an enzyme cellulolytic and xylanolytic complex is necessary. The β-(1-4) cellulose linkages are broken by cellulases, a multicomponent enzyme system composed of three major enzymes: cellobiohydrolase (exoglucanase), endoglucanase, and β-glucosidase, which act synergistically (Shi, et al., 2011). The complete saccharification of hemicellulose in a short reaction time (Xiros, et al., 2011) requires a complex mixture of xylanase, β-xylosidase, endomannanase, β-mannosidase, α-L-arabinofuranosidase, and α-galactosidase (Shi, et al., 2011; Xiros, et al., 2011) because hemicellulose nature is more heterogeneous than cellulose.
Considering tha Penicillium genus produces xylanolytic enzymes (Knob, et al., 2010; Terrasan, et al., 2010; Pedraza-Zapata, et al., 2017), in this study we proposed to use BSG as a substrate to produce an enzyme extract from Penicillium sp. HC1 and determine the effect of BSG concentration and two nitrogen sources (yeast extract and ammonium sulfate) on the activity of endoglucanase, cellobiohydrolase, β-glucosidase, and xylanase at 6, 10, and 12 days. Then we assessed the BSG enzymatic hydrolysis using the extract with the highest xylanase activity at its optimum pH and temperature for producing reducing sugars and determining the effect of BSG concentration, the dosage of xylanase, and the reaction time.
Materials and methods
Conditions for enzyme production from BSG using Penicillium sp. HC1
BSG source and characterization. The BSG supplied by Bavaria S.A. from the brewery located in Tocancipá, Colombia, was dried at 50°C to achieve 10% of humidity and then ground and sieved to obtain particles smaller than 1 mm. BSG was characterized according to the standardized methods reported by the United States National Renewable Energy Laboratory (NREL) (Hyman, et al., 2008).
Enzyme production conditions. We isolated Penicillium sp. HC1 from soils where rice-harvesting had taken place (Gutiérrez-Rojas, et al., 2012). We selected it given their cellulolytic and xylanolytic activity. The fungus was conserved on agar plates at 4°C in the biotechnology laboratory at Pontificia Universidad Javeriana (Bogotá, Colombia), then reactivated in potato dextrose agar (PDA), and incubated at 25°C for eight days. For inoculum production, the fungus was cultured in the same medium and incubated at 28°C for eight days. Conidia were suspended in 0.85% saline solution (w/v) and 0.1% Tween 80 (v/v). Its concentration was adjusted to 106 mL-1 and 2 mL of this suspension were used to inoculate liquid media for enzyme production. Cultures were prepared in 100 mL Erlenmeyer flasks charging 18 mL of a solution composed of (g L-1): 0.3 KH2PO4; 0.83 MgSO4.7H2O; 0.3 CaCl2; 0.005 FeSO4.7H2O; 1.56 mg L-1 MnSO4; 1.4 mg L-1 ZnSO4, and 0.2 mL L-1 Tween 80 (Mandels & Weber, 1969) and the mass of BSG required to obtain 1, 3 or 5% (w/v) suspensions. According to the experimental design, some cultures were not supplemented with a source of nitrogen while others were supplemented with an organic (yeast extract) or inorganic (5 g L-1 ammonium sulfate) source of nitrogen. For all the cultures the pH value was adjusted to 6.0 using a solution of NaOH (0.1N) or HCl (0.1N) as required. Cultures were incubated at 30°C at 150 rpm and taken out at different times (6, 10, or 12 days) for evaluation. The content of each Erlenmeyer flask was centrifuged at 15.300xg for 15 minutes at 7°C (Sorvall RC 6 Plus, Thermo Scientific Co., Waltham, MA, USA). We filtered the enzyme extract under sterile conditions with 0.22 urn membranes and then assessed the endoglucanase, cellobiohydrolase (exoglucanase), β-glucosidase, and xylanase activity.
Enzyme activity assessment. We determined the endoglucanase activity (E.C 3.2.1.4) in a reaction mixture containing 500 μL 2% carboxymethylcellulose (CMC) (w/v) (Sigma, St. Louis, MO, USA) prepared in 50 mM citrate buffer at pH 5.0 with 500 μL enzyme extract under appropriate dilution at 40°C for 60 minutes (Ghose, 1987). Reducing sugars were determined by the 3,5 dinitrosalicylic acid method (DNS) (Miller, 1959). A unit of enzyme activity was defined as the quantity of enzyme required to release 1 μmol reducing-sugar equivalent to glucose per minute under the test conditions.
The cellobiohydrolase activity (E.C 3.2.1.91) was determined in a reaction mixture containing 500 μL 1% microcrystalline cellulose or Avicel (w/v) (Sigma, St. Louis, MO, USA) (de Siqueira, et al., 2010) prepared in 50 mM citrate buffer at pH 5.0 with 500 μL enzyme extract under appropriate dilution at 40°C for 60 minutes. Reducing sugars were quantified by DNS. A unit of enzyme activity was defined as the quantity of enzyme required to release 1 μmol reducing sugar per minute equivalent to glucose under assay conditions.
For assessing β-glucosidase activity (E.C 3.2.1.21) we used the methodology described by Valášková & Baldrian (2006) using 5 mM p-nitrophenyl-p-D-glucopyranoside (PNPG) (Sigma, St. Louis, MO, USA) as a substrate prepared in 50 mM sodium acetate buffer at pH 5.0. The reaction mixture contained 400 μL substrate and 100 μL enzyme extract incubated at 40°C for 60 minutes; it was stopped by adding 250 μL 0.5 M sodium carbonate and the absorbance was read at 400 nm. We calculated the enzyme activity using a ρ-nitrophenol standard curve. A unit of enzyme activity was defined as the quantity of enzyme required to release 1 μmol ρ -nitrophenol per minute under assay conditions.
The xylanase activity, or endo-1,4-B-D-xylanase xylan hydrolase (EC 3.2.1.8), was determined in a reaction mixture containing a 50 μL sample and 450 μL 1% beechwood xylan (w/v) prepared in 50 mM citrate buffer (pH 5.3). We determined the enzyme activity at 50°C for 5 minutes (Bailey, et al., 1992) while for the released reducing sugars we used the DNS technique with xylose as the standard. A unit of xylanase activity was defined as the quantity of enzyme required to release 1 μmol of reducing sugar per minute equivalent to xylose under test conditions.
Statistical analysis
A randomized block experimental design was used considering two factors, three levels, and two blocks. We evaluated the factors of BSG concentration (levels:1,3 and 5% w/v) and the additional nitrogen source supplement (levels: without supplement, with an organic source, and an inorganic source at 5 g L-1). According to this design, nine experiments were performed in triplicates and evaluated at three different times: 6, 10, and 12 days of culture as shown in table 1. We analyzed the data for statistical significance using a one-way ANOVA to evaluate the association and the influence among variables with the Design-Expert V. 10 software (Stat-Ease, Inc, Minneapolis, USA). The response variables were endoglucanase, cellobiohydrolase, β-glucosidase, and xylanase enzyme activity.
Table 1 Variables and their levels employing a factorial design for Penicillium sp. HC1 enzyme production and BSG as a substrate
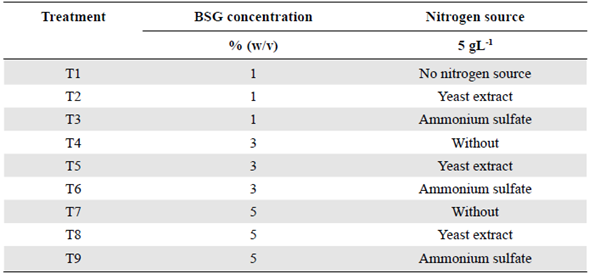
BSG treatment for hydrolysate preparation. Considering the BSG composition, the increasing interest for xylanolytic enzymes in recent years, and their potential applications in hydrolytic processes (Song, et al., 2016; de Sousa Gomes, et al., 2017; Li, et al., 2018), we selected the conditions that generated the highest xylanase (enzyme extract) production for hydrolysate preparation.
To produce enzyme extract, we used 1 L Erlenmeyer flasks containing 180 mL of the solution already described supplemented with 5 g L-1 ammonium sulfate as a nitrogen source. We added 3% BSG (w/v) to each Erlenmeyer flask and then 20 mL 106 mL-1 conidia suspension was inoculated and incubated for 10 days at 150 rpm. After incubation, we separated the enzyme extract by centrifugation at 30°C 15300xg for 20 min and then at 7°C Sorvall RC 6 Plus (Thermo Scientific Co, Waltham, MA, USA). The supernatant was filtered under sterile conditions with 0.22 um nitrate cellulose membranes. Finally, the xylanase enzyme activity was determined following the procedure already described.
Effect ofpH and temperature on the xylanase activity of the enzyme extract. The effect of pH on the xylanase activity of the enzyme extract was evaluated at a 3 to 8 pH under a constant temperature of 50 °C using the following buffers: 0.1 M sodium citrate (pH 3, 3.5, 4, 5, 5.5, 6, 6.5, and 7) and 0.1 M sodium phosphate (pH 8). The effect of temperature on the xylanase activity from the enzyme extract was evaluated at temperatures ranging from 30 to 70°C with 10°C intervals at the pH selected according to the results of the previous stage.
Reducing sugar production. The xylanase dosage and the BSG concentration effects on reducing sugar production were determined using a central compound design (CCD) determined by the Design-Expert V. 10 (Stat-Ease, Inc, Minneapolis, USA) statistical software. Thus, the previously mentioned two factors were evaluated at two levels (+1 and -1), two axial points (+1.5 and -1.5), and one central point (0) resulting in nine treatments and 29 experiments with their respective replicas (five replicas for the central point and three for the axial points and the levels). For establishing the effect of time on enzyme hydrolysis for reducing sugars production, all experiments were evaluated at six reaction times (2, 4, 6, 8, 10, and 24 h).
For the experiments, we used 100 mL Erlenmeyer flasks with 20% effective volume. The BSG mass was added according to the experiment concentration and sterilized at 121°C for 15 minutes. Next, the enzyme extract solution was diluted in citrate buffer at optimal pH and we added 20 mL sterile solution to the flasks and shook them at 150 rpm at the optimal temperature at the dosage and time established in the experimental design. The content of each Erlenmeyer flask was centrifuged at 15300 x g for 15 minutes at 7°C (Sorvall RC 6 Plus, Thermo Scientific Co., Waltham, MA, USA), and the supernatant was filtered under sterile conditions employing 0.22 um membranes. The concentration of reducing sugars and the sugar profile were determined by DNS and HPLC, respectively.
Statistical analysis
To evaluate if pH and temperature had a significant effect on xylanase activity, we used a one-way ANOVA performed in the Design Expert V. 10 software (Stat-Ease, Inc, Minneapolis, USA). Likewise, a two-way ANOVA was carried out on experimental design results (CCD) to evaluate BSG concentration, xylanase dosage, and the interaction between both factors on reducing sugar release adjusting the results to a second-order model.
Analytical methods. Reducing sugar quantification was performed using the 3,5-dinitrosalicylic acid method, also known as DNS (Miller, 1959). Concentrations were calculated by means of xylose or glucose standard curve depending on the case. Glucose, xylose, arabinose, and xylitol concentrations were determined by means of high-performance liquid chromatography (HPLC) in a Shimadzu Prominence LC-20AT (Shimadzu Tokyo, Japan) chromatograph with Shimadzu RID-10A refraction index and a Sugar Pack SZ5532 (150 mm x 1.6 mm; Shodex) column at 65°C using acetonitrile and water (80:20) as a mobile phase at 1 mL min-1 flux. All the analytical determinations were performed in triplicate.
Results and discussion
BSG characterization
The BSG we used in the study had the following composition (% w/w dry matter): 17.2±0.4 protein, 12.6±0.5 extractives, 13.4±1.0 lignin, 19.3±1.0 cellulose, 30.1±0.4 hemicellulose (20.7±0.4 xylose, 9.4±0.2 arabinose), 0.2±0.008 acetyl groups, 3.5±0.1 ashes, 3.8±0.2 other components.
Enzyme production from BSG using Penicillium sp. HC1
Under the conditions assessed in the study (Table 1), Penicillium sp HC1 produced several enzymes with BSG as a substrate. Schneider, et al. (2014) attributed enzyme production to the filamentous fungi's ability to secrete a pool of proteins, which has motivated their intensive use for industrial enzyme production. However, Penicillium sp. morphology and the mechanisms involved in cell growth and product formation are unknown.
Endoglucanase, cellobiohydrolase, β-glucosidase, and xylanase activities after 10 and 12 days under the conditions of treatments T4, T6, T7, and T8 are shown in figure 1. The data obtained under the conditions of the other treatments and after 6 days are not included as they were significantly smaller than those we report. Except for the activity of cellobiohydrolase and β-glucosidase under the T7 and T8 conditions and the xylanase activity under T6 conditions, all enzyme activities were almost the same at 10 or 12 days.
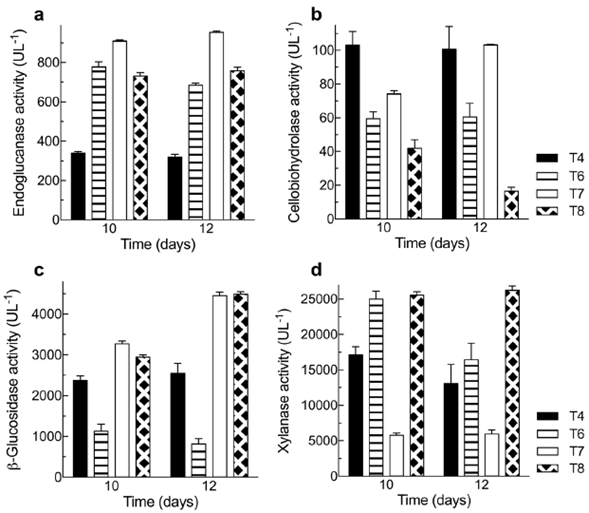
Figure 1 Maximum enzyme activities obtained from Penicillium sp. HC1 production using BSG as a substrate. (a) Endoglucanase activity. (b) Cellobiohydrolase activity. (c) β-glucosidase activity. (d) Xylanase activity. Each bar represents the average of three replicas and errors bars, the standard deviation. T4: 3% BSG (w/v) without additional nitrogen source, T6: 3% BSG (w/v) and ammonium sulfate as source of nitrogen T7: 5% BSG (w/v) without additional nitrogen source, T8: 5% BSG (w/v) and yeast extract as nitrogen source. Enzymatic activities for treatments 1, 2, 3, 5, and 9 had the lowest values for all enzymes and, thus, are not depicted in this figure.
The maximum enzyme activity for endoglucanase, cellobiohydrolase, β-glucosidase, and xylanase was 954.3±17.4 U L-1 (T7 - 12 days), 103.3±1.0 U L-1 (T7 - 12 days), 4491±169 U L-1 (T8 - 12 days), and 26233±1870 U L-1 (T8 - 12 days), respectively. Under the same treatment conditions, the reduction in endoglucanase and xylanase activities with a 10-day production time was only 4.7% and 2.5 %, respectively. The highest enzyme activity for cellobiohydrolase (T7-12 days) compared with the following lower one at 10 days (T4) rendered a reduction of only 0.2%. Therefore, for these three enzymes, the production time can be defined as 10 days. The reduction in the β-glucosidase activity with a production time reduced from 12 to10 days under T8 conditions was 34%. However, the reduction was only 27% when comparing T8-12 days with T7-10 days. The interaction analysis among variables for treatments on day 10 and 12 are shown in table 1S,https://www.raccefyn.co/index.php/raccefyn/article/view/1379/3108. BSG concentration was the variable with the highest influence for all the enzyme activities, except for cellobiohydrolase, mostly influenced by the nitrogen source. When the endoglucanase activity with no nitrogen supplementation (T7-10 days) was compared simultaneously with T8 and T9 treatments (yeast extract: 732.1 ± 52.2, and ammonium sulfate: 719.7 ± 24.6 U L-1, respectively), we observed an activity reduction of about 20%. Therefore, the addition of a nitrogen source was not necessary, which is very attractive for the industrial production of enzymes at a low cost.
The production of endoglucanase, cellobiohydrolase, β-glucosidase, and xylanase by Penicillium sp using BSG, other substrates, and different nitrogen sources has been previously reported. Pedraza-Zapata, et al. (2017) reported endoglucanase, cellobiohydrolase, and β-glucosidase activities of 83.4 U L-1 (10 days), 266.2 U L-1, and 45.9 U L-1 (8 days), respectively, when the same fungus was employed with carboxymethylcellulose (CMC) as a substrate and with no nitrogen source. In our study, the highest endoglucanase and cellobiohydrolase activities were obtained under the T7-10 day conditions with no nitrogen source. As β-glucosidase activity under the same conditions and time was 3269 U L-1, we can say that BSG is a better substrate compared with CMC for such enzyme production. Terrasan, et al. (2013) claimed they had produced xylanases, β-xylosidases, and arabinofuranosidases using Penicillium janczewskii and sugar cane bagasse, oat bran, and BSG at optimized conditions. In their study, they obtained under optimized conditions (7 days, BSG 2%) a maximum xylanase activity of 15190 U L-1. In contrast, in ours, the maximum value obtained under T8-10 day conditions was 25575 U L-1, i.e., a 68% higher activity.
Terrone, et al. (2018) reported xylanase production by Penicillium chrysogenum F-15 strain using different agroindustrial biomass: using Vogel medium supplemented with 1% (w/v) BSG for cultivation, the xylanase activity was 2280 U L-1 after 7 days at 28°C, less than 10% of that one obtained under the conditions assessed in our work. Hassan, et al. (2020) cultured Penicillium sp in basal liquid media supplemented with 1.0% (w/v) of xylan and registered a xylanase activity after 7 days of culture at 30 °C of 7400 ±0.1 U L-1, approximately 30% of the highest activity reported by us.
Besides the industrial importance of xylanases, these enzymes were particularly relevant in our study because they act on hemicellulose hydrolysis, the main component of BSG. Xylanase activity and productivity using Penicillium sp. HC1 in the three sampling times and each of the treatments proposed are shown in table 2. These results evidence that there was no statistically significant difference between T6 and T8 after 10 days. These treatments produced the highest xylanase activity, which means the enzymes can be produced with organic or inorganic nitrogen sources. The protein content in the BSG is enough to obtain a high xylanase activity but around 30% lower than that obtained with the nitrogen supplementation.
Table 2 Xylanase activity and productivity by Penicillium sp.HC1 using BSG as a substrate
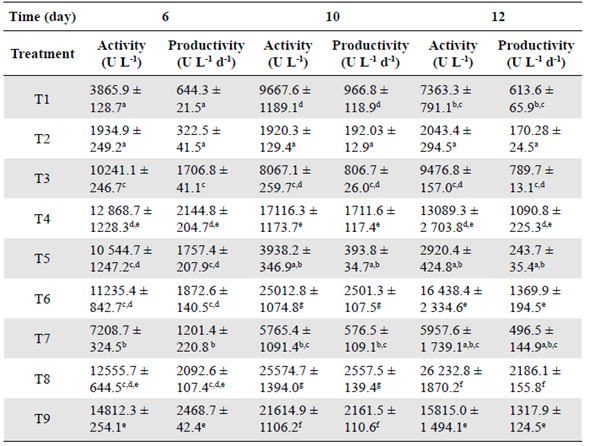
All the results are expressed as mean ± SD from three experiments (n = 3). One-way ANOVA has been conducted for each column with (p<0.05). Since analysis is significant, a post-hoc analysis (Tukey HSD test) was carried out to identify which treatment(s) were statistically different. Same letter denotes means are not significantly different (p>0.05).
When comparing T1 (1% w/v BSG), T4 (3% w/v BSG), and T7 (5% w/v BSG), all with no nitrogen source supplementation and at any of the sampling times, we noticed that the activity increased when BSG concentration was augmented from 1% w/v to 3% w/v. However, a further increase in the BSG concentration to 5% w/v had a notably negative effect. For example, on day 10, when we compared treatments 4 and 7, we observed a threefold enzyme activity decrease, which may be explained by an inefficient mass transfer or possible substrate inhibition due to an increase in substrate concentration. According to Gubatz & Shewry (2011), BSG has an important protein fraction used as nitrogen reserve, and structural proteins, including globulins, prolamins, and glutenins covering p-glucans in the grain in a cell layer known as aleurone. Increasing the BSG concentration in the media could reduce mass transfer between the catalytic sites of the xylanase enzymes with p glycosidic bonds of BSG arabinoxylan making the access of the microorganism to the substrate more difficult. Finally, Knob, et al. (2013) cultured Penicillium glabrum using the same substrate (Vogel medium supplemented with BSG 1% w/v) and the same pre-treatment (grinding) we used in our study. They reported a 42450 UL-1 xylanase activity after 6 days at 28 °C, 66% higher than the highest obtained by us.
BSG treatment for hydrolysate preparation
For this stage, we selected the conditions of treatment 6: 3% BSG (w/v), 5 g L-1 ammonium sulfate, and 10 days of processing, because the xylanase activity is similar to the highest obtained in our study (T7-10 days) but using a cheaper source of nitrogen.
Effect of pH and temperature on the xylanase activity in the enzyme extract. As for the effect of pH and temperature on the xylanase activity from Penicillium sp. HC1, experimental results showed that both variables had a significant effect (p<0.0001 for both factors. The highest activity was obtained at pH 4 (Figure 2A) and 50°C (Figure 2B), although no significant difference was observed at 40°C (p=0.1233). Xylanase characterization when it is produced by different Penicillium species and strains revealed the usual range for optimal enzyme activity between 40 and 50°C and a pH between 3.0 to 7 (Driss, et al., 2012; Liao, et al., 2012). However, some studies have reported xylanases produced at a higher temperature than the previously reported. For example, Terrasan, et al. (2016) reported that P. janczewskii produced the enzyme with a maximal activity at pH 6.0 but a temperature of 65 °C. Ouephanit, et al. (2019) found optimal xylanase activity at 55 °C and pH 5 when the xynA gene encoding xylanase A of Penicillium citrinum was successfully synthesized and expressed in Yarrowia lipolytica. Other reports described xylanases produced by the genus employed in our study with optimal temperatures below 40°C, such as those produced by Mucor sp with an optimal temperature at 30°C (Hassan, et al., 2020).
Reducing sugars production. To assess reducing sugar production by BSG enzymatic hydrolysis, we selected pH 4 and 40°C based on the previous findings. BSG was treated with Penicillium sp HC1 enzyme extract at different times between 2 h and 24 h. Reducing sugars were released from the onset of the process (2 h) progressively increasing up to 24 h when the highest reducing sugar concentrations were obtained for all the tests performed (Figure 3). The maximum reducing sugar concentration (12.5±0.15 g L1) was obtained at 6% BSG (w/v) and 1000 U g-1 of xylanase. For this test, the concentrations measured of xylose, arabinose, and glucose (g l-1) were 3.14±0.01 1.91±0.037, and 3.79±0.024, respectively. We observed a higher proportion of glucose, probably due to a multienzyme system including endoglucanase, cellobiohydrolase, and β-glucosidase (Figure 1) acting on the cellulose present in the BSG. Paz, et al. (2019) obtained diverse sugar concentrations from BSG (10% w/v) hydrolysis using an enzymatic cocktail produced by Aspergillus niger CECT 2700. They obtained 2.4 g/L xylose, 1 g/L arabinose, and 5.7 g/L glucose after 120 h. These concentrations are in the range obtained in our study but the hydrolysis time required was significantly shorter (6 h).
Figure 3 shows a fast increment of sugar concentration up to 2 h followed by a slower increment period from that hydrolysis time to 6 h. The rate of sugar increase from 6 h to 24 h was very low. Consequently, productivity at 6 h, 8 h, and 24 h decreased from 1.7 to 1.4 and 0.52 g L-1 h-1, respectively. Similar behavior was reported by Xiros, et al. (2011) using an enzymatic extract produced by Fusarium oxysporum F3 for BSG hydrolysis. Sugar concentration (xylose, glucose, arabinose, and xylobiose) notably increased up to 10 h. However, after that time no further increase in sugar concentration was observed, behavior attributed to the increasingly limited structural accessibility of cell wall matrix to enzymes as hydrolysis proceeded instead of enzyme's properties (Xiros, et al., 2011). This behavior can be explained by the inactivation of enzymes that hydrolyze biomass, although further research is required to explain the mechanism of the product inhibition, adsorption, and desorption of enzymes in the substrate surface (Shi, et al., 2011).
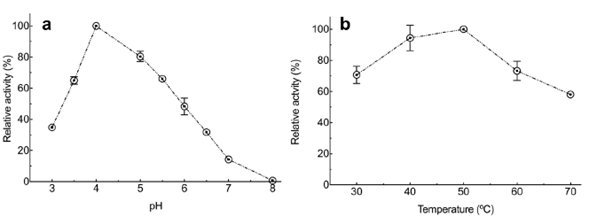
Figure 2 Effect of pH and temperature on xylanase enzyme activity from Penicillium sp. HC1 enzyme extract. (a) Enzyme activity at 50°C under different pH values. (b) Enzyme activity at pH 4 at different temperatures. Data are presented as mean ± standard deviation obtained of three replicas.
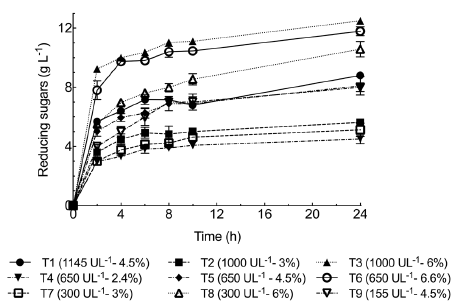
Figure 3 Reducing sugar release kinetics for BSG partial hydrolysis employing enzyme extract produced by Penicillium sp. HC1. The process was carried out at 40°C, pH 4, and 150 rpm. BSG concentrations are expressed in percentages (w/v). Data are presented as mean ± standard deviation obtained from three replicas.
Finally, Moteshafi, et al. (2016) performed BSG hydrolysis without pre-chemical treatment using enzymes produced by Bacillus subtilis D3d. They were able to produce 62.25 mg g-1 reducing sugars from BSG in 2.6 h at pH 6.12 and 50°C. For our study, the test performed at 6.6 % (w/v) BSG and 650 U g-1 of xylanase produced 153.9 mg g-1 reducing sugars after 2 h of process. It should be mentioned that this value is higher suggesting Penicillium sp. HC1 enzymes are apparently better for BSG hydrolysis. This behavior could be due to filamentous fungi's ability to secrete a pool of proteins, which has motivated their intensive use for industrial enzyme production (Schneider, et al., 2014).
Equation (1) presents the second-order model obtained for reducing sugars production, Y (g L-1), after 6 h of processing as a function xylanase doses, A (U g-1), and BSG concentration, B % (w/v). The analysis of variance (ANOVA) for that model is described in table 2S,https://www.raccefyn.co/index.php/raccefyn/article/view/1379/3108. According to the F value (182.82), the model is significant. There is only a 0.01% chance that an F-value this large could occur due to noise. Values of "Prob > F" less than 0.05 indicated that the model terms were significant. In this case, A (enzyme dosage), B (BSG concentration), AB, and B2 were significant terms for the model. The "Predicted R2" of 0.96 was in reasonable agreement with the "Adj R2" of 0.97. The "Adeq Precision" ratio of 36.85 indicated an adequate signal due to the value greater than 4. Therefore, the model was suitable to navigate the design space.
The statistical analysis revealed the BSG concentration, as well as the xylanase doses and the interaction between both factors, terms significantly affecting the release of reducing sugars during the partial BSG hydrolysis (p<0.05). However, the term with the highest incidence on the response variable was the BSG concentration, with the highest F value (832.19). Equation (1) shows that the BSG concentration was more important than the enzyme dosage. Furthermore, the interaction of factors was less important than individual factors. Therefore, the BSG concentration affected in a positive way reducing sugar concentration. On the contrary, xylanase doses resulted in a negative effect. In contrast, Garai & Kumar (2013) based on an ANOVA (quadratic model) reported that the enzyme dosage had a positive effect on reducing sugar release in an optimized saccharification model from a variety of agro-industrial residues with Aspergillus candidus-produced xylanases. Likewise, Moteshafi, et al. (2016) found xylanase doses from Bacillus subtilis D3d were the most significant factor (p<0.0001) in reducing sugar release after hydrolysis in contrast to reaction time. Figure 4 shows the response surface and contour plots of the combined effects of xylanase dosage and BSG concentration on reducing sugar production after BSG hydrolysis at 6 h.
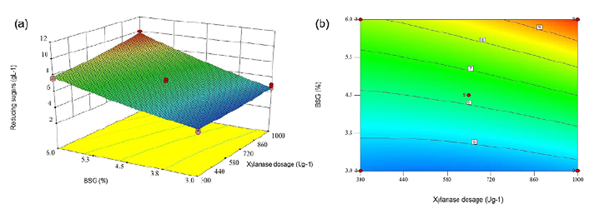
Figure 4 Response surface plot (a) and contour plot (b) of the combined effects of xylanase dosage and concentration of BSG on reducing sugars production after BSG hydrolysis of BSG in 6 h.
A numerical optimization to maximize reducing sugar concentration after 6 h of hydrolysis was performed using the Design Expert V10 software and the model presented in Equation 1. A maximum sugar concentration of 10 g L-1 was obtained using 6% (w/v) BSG and 1000 U g-1 of xylanase. To validate the model, BSG hydrolysis was performed experimentally at the predicted optimum conditions. The experimental reducing sugar concentration obtained was 10.4±0.027 g L-1, very close to the predicted one, which demonstrates the quality of the model containing xylose (2.7 ± 0.006 g L-1), arabinose (1.7 ± 0.032 g L-1), and glucose (3.3 ± 0.007 g L-1).
Conclusions
BSG is a low-cost substrate for extracellular endoglucanase, cellobiohydrolase, and β-glucosidase production by Penicillium sp. HC1 fungus using grinding as the only pre-treatment. The highest enzymatic activities with this substrate were obtained after 10 days at pH 6.0 in a culture cultivated at 30oC and 3 % (w/v) of BSG concentration. The highest enzymatic activities for endoglucanase, cellobiohydrolase, and β-glucosidase were obtained without adding any nitrogen source but supplementation with ammonium sulfate or yeast extract was required to increase the xylanase activity. Based on our results, the enzymatic extract obtained hydrolyzes BSG producing xylose, arabinose, and glucose. The concentration of glucose was the highest because the enzymatic extract contains enzymes that hydrolyze cellulose. A statistical quadratic model represented adequately the relationship between reducing sugar production, enzyme dosage, and BSG concentration after 6 h of hydrolysis. The highest reducing sugar concentration was obtained at pH 4 and 40°C using 6% BSG (w/v) with a dosage of 1000 Ug-1 dry BSG of xylanase in the Penicillium sp HC1 enzyme extract. The predicted value for this variable was like the one obtained experimentally, which validated the model.