1. Introduction
The concern to reduce dependence on fossil fuels, as well as to reduce pollution caused by the emission of greenhouse gases (GHGs) has stimulated the development of studies on the production of fuels from renewable resources [1,2]. In this sense, biofuels are considered promising alternatives, as they have a chemical composition similar to traditional fossil fuels and low pollutant emissions [2,3].
Biofuels can be obtained from different sources of biomass, including cellulosic biomass, biomass derived from sugar and starch, and biomass based on triglycerides [1,2]. Biomass based on triglycerides includes edible vegetable oils [4,5], used vegetable oils [6], animal fat [7], and non-edible vegetable oils [8,9], and can be converted into biofuels via thermal cracking [10,11] or catalytic cracking (also known as thermal catalytic cracking) [12-20], which is a simple and economical method with products that resemble petroleum fuels [21-23].
Several types of biomass based on triglycerides have been used for the production of biofuels through cracking [4-6,8,14,24]. Among the biomasses used for this purpose, it is possible to highlight the palm oil tree (Elaeis guineenses, Jacq.), “dendezeiro” (in Portuguese), which is an oilseed with a high yield of crude palm oil per hectare [25].
Thermal catalytic cracking products of biomass based on triglycerides include gas, organic liquid product (OLP), coke, and water [26-28]. The OLP consists of oxygenated compounds and hydrocarbons, which correspond to the boiling-point range of gasoline, aviation kerosene and diesel [9,27,29-31]. Some components, such as oxygenated compounds, confer undesirable characteristics to the OLP, limiting its use as a direct substitute for liquid transport fuel [32-34]. Therefore, various types of distillation, including atmospheric pressure distillation, has been applied to the fractionation and upgrading of OLP [20,31,33,35-43] in order to provide biofuels with better physical-chemical property values than those presented by the feed or within the limits specified by regulatory agencies of each country [42]. Due to the complex composition of the OLP, its boiling point starts below 100 °C and reaches the range of 250-280 °C [44]. Atmospheric distillation has the advantages of being an uncomplicated and mature separation process widely used in the petrochemical industry, presenting itself as a technical and economically viable way to separate the complex composition of OLP into biofuels similar to gasoline, aviation kerosene and diesel [3].
Capunitan and Capareda [33] performed fractional distillation of bio-oil under atmospheric conditions and reduced pressure (vacuum). The results showed high yields of heavy fractions and a significant reduction in humidity and acid value. The chemical composition analysis showed that the aromatic and oxygenated compounds were distributed in the light and medium fractions (15-20%), while the phenolic compounds were concentrated in the heavy fraction (53%). The distillation process was effective in separating the components and producing a heavy fraction with improved properties and composition and which could be further utilized as feedstock for future upgrading procedures or as a blending material with other liquid fuels. Ferreira et al. [43] investigated the deacidification of OLP by laboratory-scale fractional distillation using columns of different heights, with and without reflux, and at the pilot scale. Biofuels (distillates) showed yields at the laboratory scale with and without reflux ranging from 62.15 to 76.41 wt % and from 71.65 to 89.44 wt %, respectively, and 32.68 wt % at the pilot scale. For pilot-scale distillation experiments, the acid values of the gasoline, kerosene, and light diesel fractions were 0.33, 0.42, and 0.34 mg KOH/g, respectively. The GC-MS results of the OLP showed 92.84% (by area) of hydrocarbons and 7.16% (by area) of oxygenates. The light diesel fraction contained 100% hydrocarbons with an acid value of 0.34 mg KOH/g, proving the feasibility of OLP deacidification by fractional distillation.
In this context, the objective of the present study was to investigate the influence of the fractional distillation process of OLP on yield and quality of biofuels similar to petroleum products such as gasoline, aviation kerosene, and diesel fuel.
2. Materials and methods
2.1. Organic liquid product
In this study, the feedstock used was an OLP obtained by thermal catalytic cracking of crude palm oil at a pilot plant using 20 wt % of sodium carbonate (Na2CO3) as the catalyst, as described by Mota et al. [20]. These authors characterized the OLP using physical-chemical and FTIR analyses.
2.2. Experimental apparatus and procedure
The OLP was distilled into three fractions (green gasoline, green aviation kerosene, and green diesel) using the experimental apparatus described by Mota et al. [20].
OLP was submitted to laboratory-scale fractional distillation using a Vigreux Column with three stages. To obtain three fractions, the OLP was distilled within the following distillation temperature ranges: 90-160 °C (green gasoline); 160-245 °C (green aviation kerosene); and 245-340 °C (green diesel). The distillation temperature intervals were reported to give hydrocarbon fractions with similar properties to particular petroleum products.
The fractional distillation of OLP aimed to obtain the fractions previously defined for physical-chemical characterization and analysis of composition.
The OLP was heated gradually to first distillation temperature range (90-160 °C). The distilled fraction was collected in a 250-mL separation funnel and stored in amber glass bottles. After obtaining the first distilled fraction, the temperature controller of the heating mantle was adjusted to reach the second distillation range (160-245 °C). The same procedure was followed to obtain the remaining fraction (245-340 °C). At the end of the experimental procedure, there were three distilled fractions and a bottoms product.
Eq. (1) was applied to determine the yield of the distilled fractions (green gasoline, green aviation kerosene, and green diesel), as well as the bottoms product.
where m DF is the mass of distilled fraction (g), and m OLP is the mass of OLP (g).
2.3. Characterization of the distilled fractions
2.3.1. Physical-chemical properties
Distilled fractions were physically-chemically characterized according to the official AOCS and ASTM methods for acid value (AOCS Cd 3d-63), saponification value (AOCS Cd 3-25), specific gravity at 20 °C (ASTM D854), refractive index (AOCS Cc 7-25), kinematic viscosity at 40 °C (ASTM 446 and ASTM D2515), flash point (ASTM D93), corrosiveness to copper (ASTM D130), FFA content (AOCS Ca 5a-40), carbon residue (ASTM D4530) and ester value, which is the difference between the saponification value and the acid value, as described by Paquot [45].
2.3.2. FTIR spectroscopy
Distilled fractions were analyzed by FTIR spectroscopy (Shimadzu, model: Prestige 21). The absorbance spectra were obtained within the interval 4000-400 cm−1 at a resolution of 16 cm−1 using a KBr window. The samples were dropped onto the KBr surface by micropipette in order to spread the liquid and produce a uniform layer.
2.3.3. Distillation curve
The distillation curves of the distilled fractions were obtained according to the official method (ABNT/NBR 9619) using an automatic distillation apparatus (Tanaka, model: AD6).
2.3.4. GC-MS analysis
The GC-MS analysis of the distilled fractions was performed using a gas chromatograph coupled to a mass spectrometer (Shimadzu, model: GCMS-QP2010 Plus), as described by Mota et al. [20]. The relative content of compounds in the distilled fractions was calculated by the ratio of their peak area to the total peak area of the GC-MS spectra.
3. Results and discussion
3.1. Influence of fractional distillation on the yield of biofuels
According to Table 1, the OLP produced by thermal catalytic cracking of crude palm oil with 20 wt % of Na2CO3 generated three fractions after being subjected to a fractional distillation process at the following distillation temperature (DT) ranges: 90 ≤ DT ≤ 160 °C (green gasoline), 160 ≤ DT ≤ 245 °C (green aviation kerosene), 245 ≤ DT ≤ 340 °C (green diesel). Table 1 also shows that as the DT range increased, the yield of the distilled fractions increased, indicating that cracking of crude palm oil with Na2CO3 favored the production of heavier fractions such as green aviation kerosene, green diesel, and bottoms product. Weber et al. [36] obtained similar results, performing the thermal degradation of animal fat in a pilot-scale plant at 410-450 °C using a moving bed of sodium carbonate as the catalyst and 5 wt % of water, obtaining OLP. The authors also conducted a fractional distillation of the OLP produced, yielding 66 wt % of diesel fraction and 21 wt % of gasoline fraction.
The total yield from the sum of the three fractions (green gasoline, green aviation kerosene, and green diesel) was 60.43 wt %, which is higher than those found in the literature. Chew & Bhatia [46], for example, investigated the effect of additives (HZSM-5 in different Si/Al ratios, beta zeolite, SBA-15, and AlSBA-15) mixed physically with Rare Earth-Y (REY) as a catalyst in the catalytic cracking of crude and used palm oil to produce biofuels. The best results in terms of yield showed that OLP consisted of 59.3 wt % and 55.3 wt % of fractions in the boiling-point ranges of gasoline, kerosene, and diesel oil for crude and used palm oil, respectively, both using HZSM-5 (Si/Al ratio = 40) with REY as catalyst.
The bottom product was the product of the fractional distillation of OLP that showed the highest yield in the present study. The bottom product contains a wide variety of valuable chemicals, making its use possible in resins, agrochemicals, fertilizers, emission control agents [33], fuels [47], carbon anodes, steel carburization, and graphite synthesis [48].
Therefore, the results show that OLP from the thermal catalytic cracking of crude palm oil with 20 wt % Na2CO3 at 450 °C and 1 atm and at pilot scale yielded a higher amount of distilled fraction when compared to cracking OLP using commercial catalysts such as zeolites, and the bottom product formed in the fractional distillation process of the former OLP can find several applications.
3.2. Influence of fractional distillation on the quality of biofuels
3.2.1. Physical-chemical properties
As shown in Table 2, most of the values concerning the physical-chemical properties of the green gasoline fraction were considerably lower than those of the OLP. These values were also lower than those found by Xu et al. [35] and Wisniewski Jr. et al. [40] for gasoline fractions.
Table 2 Physical-chemical properties of the green gasoline fraction. FFA, free fatty acids; max, maximum; min, minimum; ANP: Agência Nacional do Petróleo, Gás Natural e Biocombustíveis, Resolution N° 40 (Specification of regular gasoline/Type A) a viscosity at 20 °C
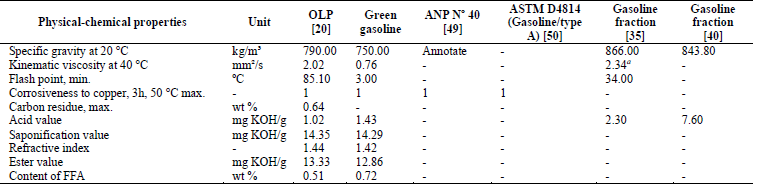
Source: The authors.
Table 3 shows that most of the physical-chemical properties of the green aviation kerosene fraction had values equal to or lower than those relating to OLP. Although the acid value did not reach the limit established by the ANP Nº 37 [51] and ASTM D1655 [52], the value obtained for this property was 1.68 mg KOH/g. This value is relatively low and can be further reduced by applying separation processes such as liquid-liquid extraction and adsorption [43,55] or even fractional distillation [43] in order to achieve the values specified by ANP and ASTM.
Table 3 Physical-chemical properties of the green aviation kerosene fraction.FFA, free fatty acids; max, maximum; min, minimum; ANP: Agência Nacional do Petróleo, Gás Natural e Biocombustíveis, Resolution Nº37 (Specification of Aviation kerosene). a Density at 15 °C, kg/m3. b Viscosity at 20 °C, mm2/s (max.) c Copper strip, 2 h at 100 °C (max.) d ASTM Test Method: ASTM D3242
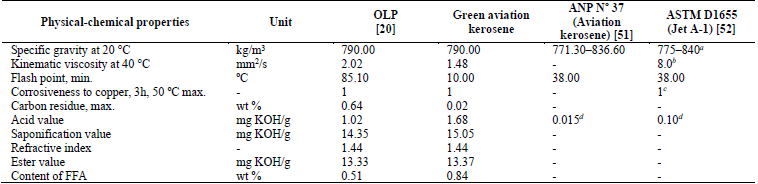
Source: The authors.
The flash point value was lower than that established by ANP Nº 37 [51] and ASTM D1655 [52], as shown in Table 3. This fact is due to the presence of low-molecular-weight compounds and a lack of an adequate percentage of normal paraffinic and aromatic compounds, resulting in a lower flash point for the green aviation kerosene fraction. The flash point indicates the presence of volatile components in the oil and is used to evaluate the overall flammability hazard of a material. The lower the flash point, the higher the concentration of light hydrocarbons in the material. This fact is corroborated in Section 3.2.4, which quotes the results of chromatographic analysis of the distilled fractions. Also, the specific gravity of the green aviation kerosene was in agreement with the specifications established for aviation kerosene according to ANP Nº 37 (Specification of Aviation Kerosene) [51] and ASTM D1655 (Jet A-1) [52].
Concerning Table 4, most of the values of the physical-chemical properties of the green diesel fraction are higher than those found for the OLP. However, the specific gravity and acid value of this fraction are lower than those found by Xu et al. [35] and Wisniewski Jr. et al. [40]. The values of properties such as specific gravity at 20 °C, kinematic viscosity at 40 °C and corrosiveness to copper of the green diesel fraction are within limits established for the Diesel S10 specification of ANP Nº 65 [53]. Properties such as flash point and acid value were not within limits established by that regulatory agency; however, the flash point was lower than that established by ANP Nº 65 [53] and ASTM D975 [54], which is due to the presence of low-molecular-weight compounds, a lack of an adequate percentage of normal paraffinic and aromatic compounds, as corroborated in Section 3.2.4.
Table 4 Physical-chemical properties of the green diesel fraction. FFA, free fatty acids; max, maximum; min, minimum; ANP, Agência Nacional do Petróleo, Gás Natural e Biocombustíveis, Resolution Nº 65 (Specification of Diesel S10). a Viscosity at 20 °C. b ASTM Test Method: ASTM D524.
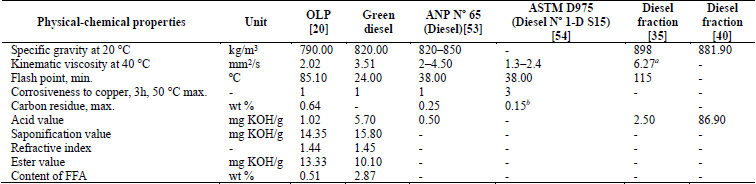
Source: The authors.
The flash point of a fuel is important for safety, as its regulation is necessary for legal and safe handling and storage [56]. The removal of volatile components by upgrading can quickly improve the flash point [39,57,58], making it possible for distillate fractions, especially green aviation kerosene and green diesel, to reach the limits set by regulatory agencies.
The acid value of the green diesel fraction does not reach the limit established by ANP Nº 65 [53], and the value, 5.70 mg KOH/g, is higher than that of the other distilled fractions, a result of the higher concentration of carboxylic acids in the green diesel fraction compared to the other fractions, as indicated in Table 5.
3.2.2. FTIR spectroscopy
The FTIR spectra of the distilled fractions shown in Fig. 1 indicates the presence of bands characteristic of saturated hydrocarbons, such as characteristic bands of the axial deformation of CH within the region 3000-2840 cm-1, corresponding to hydrocarbons from normal alkanes. Associated with these bands, we verified the presence of a band at 1375 cm-1 relating to angular deformation of methyl group C-H [59], confirming of the presence of aliphatic hydrocarbons. It is also important to note that the spectra also show broad bands in the range 3077-2750 cm-1, associated with the bands 1716 cm-1 and 1722 cm-1, which confirm the presence of carboxylic acids, aldehydes, and ketones.
3.2.3. Distillation curve
Figs. 2, 3, and 4 show the distillation curves of the fractions. In Fig. 2, the curve of the green gasoline fraction shows similarity to the standard distillation curve for regular gasoline (Type A) specification of ANP Nº 40 [49]. The curve shows that the DT exceeds the standard values for recovered volumes of 10% and 50%. However, the temperatures for other recovered volumes (90% and 100%) are consistent with the standard distillation curve for regular gasoline (Type A) from petroleum, according to the specification of ANP Nº 40 [49]. Wiggers et al. [31] obtained similar results to those of this study when fractionating soybean bio-oil into light distilled fractions, with similar distillation curves to those of gasoline A from petroleum.
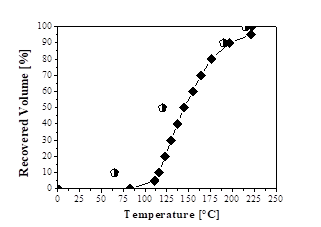
Source: The authors.
Figure 2 Distillation curves: ♦ Green gasoline fraction; Regular gasoline specification (ANP Nº 40).
Fig. 3 indicates that the green aviation kerosene fraction reached the limits for the distillation curve of aviation kerosene according to ANP Nº 37 [51]. Ertas and Alma [60] obtained simulated distillation curves for the kerosene fraction from the bio-oil of laurel (Laurus nobilis L.) and found that they could use it as fuel when blended with commercial petroleum products.
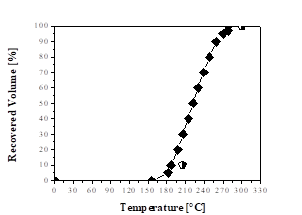
Source: The authors.
Figure 3 Distillation curves: ♦ Green aviation kerosene fraction; Aviation kerosene specification (ANP Nº 37).
Fig. 4 indicates that the experimental distillation curve of the green diesel fraction is different from the standard distillation curve in Diesel S10 specification (ANP Nº 65) [62] until a recovered volume of approximately 40%. In the range of 0-40% recovered volume, the DTs are above those established by ANP, indicating that there is an excessive concentration of heavy hydrocarbons in the green diesel fraction when compared to petroleum diesel. In contrast, the temperatures of 50% and 95% recovered volume are consistent with those established for the standard distillation curve. Ertas and Alma obtained simulated distillation curves for the diesel fraction from the bio-oil of laurel (L. nobilis L.) and also concluded that it could be used as fuel when mixed with petroleum products.
3.2.4. GC-MS analysis
The results of GC-MS analysis are summarized in Fig. 5 and Table 5. Fig. 5 shows the presence of C8-C20 hydrocarbon chains in the three distilled fractions. However, each distilled fraction has a specific hydrocarbon range. The green gasoline fraction was composed of hydrocarbons with chains in the C8-C17 range, with higher concentrations of C10-C12 hydrocarbons. According to Farah [61] and Speight [62], gasoline consists of hydrocarbons ranging from C4 to C12. Therefore, the green gasoline fraction is consistent with the limits established in the literature of the number of carbons present in the hydrocarbon chains.
The green aviation kerosene fraction consisted of C10-C19 hydrocarbons, in which the predominant hydrocarbons were the C11-C16 range. These results are consistent with those described by Speight [62], who reported that kerosene-type jet fuels (JP-4) are characterized by the hydrocarbons in the C4-C16 range. Finally, the green diesel fraction contained hydrocarbons chains in the range C13-C20, with the majority in the C15-C18 range. Speight [62] reports that the carbon number limit for diesel fuel is C8-C18.
According to Table 5, the chemical distributions of the classes of hydrocarbons and oxygenated compounds of the three distilled fractions were very different from those of the OLP produced in a previous study by Mancio et al. [13]. In OLP, hydrocarbons were abundant relative to oxygenated compounds. Similarly, Table 5 also shows that all the biofuels produced in the present study in the form of distilled fractions contained more hydrocarbons than oxygenated compounds. The fractions with the most significant hydrocarbon content were green gasoline and green aviation kerosene, with lower contents of oxygenated compounds in these fractions. Among the identified and quantified hydrocarbons were normal paraffinic, olefinic, naphthenic, and aromatic compounds, which according to Farah [61] and Szklo [56] are the main components present in the distilled fractions of crude oil. Regarding the content of aromatic compounds, the green gasoline fraction follows ANP Nº 40 [49], which states that regular gasoline must not contain more than 25 wt % of aromatic compounds in its composition.
The green diesel fraction contained the highest levels of oxygenated compounds of all three distilled fractions (See Table 5). It is to be expected that some oxygenates are not completely converted into hydrocarbons, since during triglyceride cracking reactions one of the dominant steps is the elimination of heavy oxygenated hydrocarbons such as carboxylic acids, aldehyde, ketones, and esters [63]. Among the oxygenated compounds, alcohols and ketones represent the two largest chemical families in the green diesel fraction. According to Oasmaa [57], the acidity of bio-oils arises from their content of oxygenated compounds, carboxylic acids being the class that promotes higher acidity. For this reason, the green diesel fraction, with 0.98 wt % of carboxylic acids, is the distilled fraction with the highest acid value, as shown in Table 4.
Therefore, the results of the GC-MS analysis clearly show that most of the distilled fractions can be used as energy sources by their application as bio-additives to fuels, due to their high hydrocarbon content and low levels of oxygenates. Such use of distilled fractions as bio-additives may result in emulsification of the fraction with their respective oil derivatives. Besides, the distilled fractions can be used as feedstock for further upgrading process in a crude oil refinery by hydrotreating processes [33].
It is important to note that the green gasoline fraction shows characteristics that could be improved, as naphthenic and aromatic compounds are present in low concentrations. According to Farah [61], these compounds influence the resistance to detonation, since their presence is favorable for the antiknock characteristics of fuels, such as gasoline, used in spark-ignition engines. However, the presence of normal paraffins implies a greater stability or resistance to oxidation, and hence greater durability of the fuel.
The presence of oxygenates in the green gasoline fraction can influence its volatility as well as its energy efficiency. According to Farah [61], oxygenates reduce the volatility of gasoline, improving the time of ignition or burning of fuel. According to Szklo [56], the presence of oxygenated compounds increases the latent heat of vaporization, i.e., the energy required to vaporize the liquid fuel.
Unlike the results obtained for the green gasoline fraction, the presence of aromatic compounds was not identified in the green aviation kerosene or green diesel fractions, resulting in the higher levels of other constituents, as seen in Table 5. According to Farah [61], the presence of paraffinic and naphthenic compounds, as well as the absence of aromatic compounds, in diesel derived from petroleum contributes to a good quality fuel. These characteristics are favorable because the presence of aromatic compounds in fossil fuels promotes a higher resistance to detonation in diesel combustion engines, and the presence of normal (linear) paraffinic promotes greater stability, i.e., resistance to oxidation. On the other hand, the presence of olefins results in a low freezing point and a high cetane number. The high content of olefins can be reduced by hydrogenation: typically, simple hydrogenation or non-destructive hydrogenation, which are used to improve product quality without causing a significant change in boiling-point range [58].
According to Table 5, the results of this study are similar or superior to those reported in the literature. The hydrocarbon contents of the green gasoline and green aviation kerosene fractions, for example, are significantly higher than those present in the light, middle and heavy fractions obtained by fractional distillation in the temperature ranges of ≤100 °C, 100-180 °C, and 180-250 °C, respectively, as described by Capunitan and Capareda [33]. The green gasoline also showed a hydrocarbon content higher than that obtained by Wisniewski et al. [40], who despite a DT range of <200 °C obtained a light bio-oil fraction with only 60.06 wt % of hydrocarbons from the reactive distillation. On the other hand, the green gasoline showed a hydrocarbon content similar to that obtained by Zhao et al. [64], who obtained a distilled fraction with 91.97% of hydrocarbons from the simple distillation at the similar temperature range of <200 °C. The green aviation kerosene and green diesel fractions obtained in the present study also showed very similar hydrocarbon contents to those described by da Silva Almeida et al. [41] and da Silva Almeida et al. [14] for kerosene and light diesel fractions obtained by fractional distillation at 175-235 °C and 235-305 °C, respectively.
4. Conclusions
The fractional distillation of OLP provides biofuels with most physical-chemical values within limits established by national and international regulatory agencies and with experimental distillation curves similar to standard curves. Besides, a single fractionation of OLP generated biofuels (green gasoline, green aviation kerosene, and green diesel) with higher contents of hydrocarbons than oxygenated compounds and contained hydrocarbons characteristic of the respective petroleum derivatives. Therefore, biofuels have a promising potential for use as novel products in the area of renewable energy, allowing the current consumer market to purchase products that can partially or entirely replace petroleum products, as do bioethanol and biodiesel.