1 Introduction
Dyes are organic compounds with complex molecular structures and high molecular weights, which can give color to a substrate, using selective absorption of wavelengths in the light spectrum, without being affected by physical (e.g. light, sunshine, wetness), biological (e.g. microorganisms from the environment) or chemical factors (e.g. detergents) [1]. Color from dyes is imparted by the presence of chromophore groups, usually aromatics, that consists of a conjugated pi-system, alternating single and double bonds; such aromatic groups can contain nitro functional groups, amines, hydroxyls, among other substituents [2,3].
The first dyes were obtained by extraction from animal and vegetable cells, and minerals. In 1856, William Perkin obtained the first chemically synthesized dye; it was named mauveine [4]. This was a milestone in the chemical industry that triggered a great number of investigations searching for new synthetic dyes, with more stability to light and water than natural dyes.
As a result, since then, the market of dyes has had a sustained increase. In 1930, 210,000 tons of dyes per year were produced; in the 60s, 346,000 tons per year, and for the mid-70s decade, more than 750,000 tons per year [5]. At the beginning of the XXI century, around 10,000 synthetic dyes had been developed [6] and their production reached 800,000 tons per year [7,8]. The above mentioned is a result of the notable advantages that synthetic dyes have, compared with natural dyes, for instance: low production cost, high stability, a wide range of colors, and easier application. These advantages have propitiated their use in many industries including paper, plastic, textile, rubber, cosmetics, pharmaceutical products, and foods; they are also employed in photography and petroleum additives [9,10].
The textile industry is the main consumer of synthetic dyes, for the coloration of its materials. In these processes, 10-15% of colored waters are lost to effluents owing to the inefficiency of the dyeing process, which is 200,000 tons per year flowing to water bodies [11]. As previously stated, synthetic dyes are very stable, becoming an important environmental problem due to their long permanence in the environment and their low biodegradability [7,12,13].
Synthetic dyes significantly affect the photosynthetic activity of the organisms living in the water, because of the reduction of the light that can penetrate through it [14]. On the other hand, they can result toxic, mutagenic, carcinogenic or teratogenic for the aquatic fauna and flora [15], as a consequence of the presence of aromatic amines, chlorides and heavy metals such as chromium, cobalt, nickel, and copper in their structure. Besides, as a result of the breaking of their structure, the generation of other compounds that on certain occasions are more toxic than the initial ones is possible. By way of illustration, as from azo dyes, toxic amines could be released, because of the breaking of the azo reactive group [16].
Diverse physical and chemical processes for dyes removal from wastewaters exist. Among the physical methods are adsorption, flocculation, coagulation, and membrane filtration [8,17-19]. Chemical methodologies are mainly based on the oxidation with hydrogen peroxide, Fenton's reaction, photocatalysis, electrochemistry, and ozone [20-23]. Both, chemical and physical processes, have operational problems and higher costs; besides, they present low effectiveness, limited versatility, interference by other constituents of the wastewaters, and difficulties with the management of generated residues; therefore, their implementation at the industrial level is limited [1,24,25].
Over the past few decades, biological methods as an effective and viable alternative for the solution of the problem engendered by synthetic dyes have been proposed. These methods draw upon bioremediation, a process that employs microorganisms to catalyze the complete degradation or transformation of toxic compounds toward less harmful forms and environmentally friendly [26]. White-rot fungi (WRF) are the most studied microorganisms owing to their capacity to disintegrate lignin, which has a similar structure to the dyes [27]. WRF use mostly two strategies to break down dyes: the action of their ligninolytic enzymes such as laccase (Lac), manganese peroxidase (MnP) and lignin peroxidase (LiP), and the biosorption of the dye [28,29]. The general mechanism employed by ligninolytic enzymes entails the production of free radicals responsible for a series of complex reactions resulting in the breaking of the dyes [30]. Biosorption is an uptake phenomenon of chemical substances (contaminants) from an aqueous solution by biomass, through physical-chemical mechanisms [31,32]; the term refers to a number of processes independent of the metabolism that essentially take place at the cell wall [33], which consists of polysaccharides, proteins and lipids that offer diverse functional groups that can interact in different ways with the dyes [34,35]. It constitutes a promissory alternative for detoxification of wastewater, due to its good capacity to eliminate pollutants and to the abundance of raw materials (marine algae, bacteria, the biomass of fungi or residues from industrial activities) that can be used as adsorbents [33].
Leptosphaerulina sp. is an ascomycete fungus with the capability to break down synthetic dyes, mostly through its laccase enzyme [36,37], such enzyme can be constitutively produced or induced by using phenolic compounds and compounds as copper sulfate, 2,5-dimethylaniline and ethanol, among others [36,38]. Even though the production of laccase enzyme has been already reported in species from the genus Leptosphaerulina [39], its use in the treatment of toxic compounds, like dyes, has been scarcely reported [36,37,40]. Sajben-Nagy et al. (2014) characterized an extracellular laccase from Leptosphaerulina chartarum with the capacity to degrade xenobiotic compounds in vitro [41] and Copete et al. (2018) used enzymatic extracts of Leptosphaerulina sp. so as to treat wastewater contaminated with antibiotics [25] showing the ability of this fungus to remove diverse contaminants. Besides its enzymatic capacities, Leptosphaerulina sp. has also exhibited the ability to remove azoic dyes by adsorption mechanisms [37].
Turquoise Blue (TB), also known as Corazol Turquoise Blue or Reactive Blue 21, consists of a tetrasulfonated copper-phthalocyanine with vinyl sulfone reactive groups that make the dye more water-soluble (Fig. 1a). It is a desirable property that allows it to be broadly used in the textile industry in order to dye clothes and as impression colorant [42,43]. However, TB presence into wastewater is also an environmental problem.
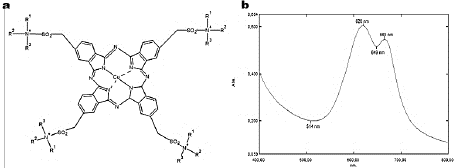
Source: The authors.
Figure 1 Turquoise Blue. (a) Dye chemical structure, and (b) Absorption spectrum.
In this research work, the potential of Leptosphaerulina sp. Colombian native fungus and its enzymatic extracts to decolorize aqueous solutions of TB, was determined and improved. To do this, an experimental design was developed where four operational conditions were controlled: nutrients availability, pH, temperature and the presence of inductors in the culture broth. The effects of such factors were evaluated using response surface methodology, in order to establish the more favorable conditions for decoloring the dye and for obtaining the higher enzymatic activities.
2 Material and methods
2.1 Chemicals
Turquoise Blue (TB, phthalocyanine dye, CAS 85650-98-2, λmáx= 620 nm) (Colourtex) (Fig. 1a), donated by Fabricato -Tejicondor S.A. a Colombian textile industry. Glucose, malt extract, yeast extract and peptone were obtained from Carlo Erba. 2,2'-azino-bis (3-ethylbenzothiazoline-6-sulphonic acid) (ABTS) was bought from Sigma - Aldrich. Ethanol, methanol, butanol, acetone and chloroform were purchased from Merck. Copper sulfate, Ammonium L-(+)-tartrate and 2,6-dimethoxyphenol (DMP) were acquired from Alfa Aesar.
2.2 Microorganism and culture conditions
Leptosphaerulina sp. was isolated from lignocellulosic material in El Valle de Aburrá (Medellín, Colombia) and conserved in the collection of microorganisms of PROBIOM (CECT 20913) research group [36,40]. The fungus was maintained in malt extract agar at 4 °C until use. Mycelium from 8- days-old culture was homogenized and used as inoculum in the degradation process [36]. For degradation assays, a culture medium (pH 5.6) containing 10 g L-1 glucose, 1 g L-1 yeast extract, 5 g L-1 peptone, 2g L-1 ammonium tartrate, 1 g L-1 KH2PO4, 0.5 g L-1 KCl, 0.5 g L-1 MgSO4. 7HO and 1 mL of a mineral solution that contains per liter: 100 mg Na2B4O7. 10H2O, 70 mg ZnSO4. 7H2O, 50 mg FeSO4. 7H2O, 10 mg M11SO4. 7HO and 10 mg (NH4)6Mo7O24. 4H2O [44] was used. This work was authorized by the Autoridad Nacional de Licencias Ambientales (ANLA) under the research permit No. 8 de 2010 (Resolución 324 de 2014) and the Ministerio de Ambiente y Desarrollo Sostenible of Colombia with the agreement No. 96 of 2014 to genetic resources access.
2.3 Bioprocessfor decolorization of wastewaters colored with TB
The methodological approach taken in this study was based on an experimental central composite design of response surfaces.
Table 1 Experimental design to evaluate TB removal by Leptosphaerulina sp. at 25 °C, 30 °C and 35 °C, t= 15 days.
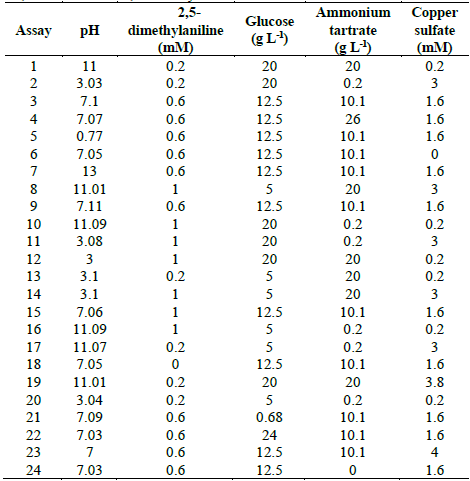
Source: The authors.
Five variables were examined: pH (3-11), 2,5-dimethylaniline (0-0.6 mM), glucose (0.68-20 g L-1), ammonium tartrate (0-26 g L-1), and copper sulfate (0-3.8 mM). This gave place to 24 experimental assays (Table 1). The experimental design of Table 1 was executed at three different temperatures (25 °C, 30 °C and 35 °C). All experiments were carried out in Erlenmeyer flasks of 250 mL containing 60 mL of culture broth supplemented with TB 200 at mg L-1 as reported in previous works [37,40]. Flasks were inoculated with 3 mL of mycelium previously homogenized in a sterilized blender at 8000 rpm for 60 s and later incubated at 28 °C and 200 rpm for 15 days. Response variables were decolorization percentage and enzymatic activities (Lac, MnP and LiP).
2.3.1. Dye decolorization determination
Dye decolorization was measured spectrophotometrically at 620 nm (Fig. 1b), with a Shimadzu UV-1800 scanning equipment and was expressed in terms of decolorization percentage (D%), by using the eq. (1) [45]:
Where, A0 corresponds to the initial absorbance and At is the absorbance at the t sampling time.
2.3.2. Enzymatic activities
Lac activity was spectrophotometrically determined (Shimadzu UV-1800) by measuring the oxidation of 3 mM ABTS in 0.1 M sodium tartrate buffer pH 3.0 (8420, 36,000 M4 cm-1). MnP activity was estimated by measuring the oxidation of 1 mM DMP in 0.1 mM sodium acetate buffer pH 4.5 (8469, 27,500 M-1 cm-1). LiP activity was determined using the 2 mM veratryl alcohol oxidation in 0.1 M sodium tartrate buffer pH 3.0 (8310, 9300 M-1 cm-1) [44,45]. All the enzymatic activities were reported as the amount of enzyme required for oxidizing 1 umol of the substrate in 1 min (U units).
2.3.3. Determination of TB dye sorbed into fungal biomass
To establish the contribution of the biosorption mechanism in the decolorization process of TB, some solvents were used as proposed by Robinson et al. (2002) [46]. Briefly, independent experiments (one for each solvent and combination of solvents) were carried out growing Leptosphaerulina sp. in the optimized conditions for TB decolorization found at the present work. The biomass with the sorbed dye was put in contact with one of the following organic solvents: ethanol, methanol, butanol, acetone or chloroform. The solvents were added in concentrations of 30%, 50%, 70% and the maximum concentration from the solvent supplier. In addition, mixtures ethanol-methanol (1:1) and ethanol-butanol (1:1) were employed. The extracted dye was spectrophotometrically quantified at 620 nm, as previously described. In the case that the solvents did not desorb the dye from fungal biomass, an indirect determination of the sorbed TB was carried out. The following indirect determination of the sorbed dye was posited: Leptosphaerulina sp. was grown in Erlenmeyer flasks of 250 mL containing 60 mL of culture medium (Section 2.2) without dye, at 200 rpm for 8 days. The biomass obtained at the end of the culture was harvested and weighed. This biomass was subjected to heat treatment (121 °C, 15 psi, 20 min) to denature any protein present in the samples and to prevent the production of new enzymes. Consecutively, the biomass was re-suspended in culture medium with TB (200 mg L-1) and was kept at 28 °C and 200 rpm, for 8 days. Finally, TB decolorization by biosorption mechanism was spectrophotometrically determined measuring the difference between the decolorization reached with the active biomass and the biomass subjected to heat treatment (inert biomass).
3 Results
3.1 Evaluation of wastewater decolorization dyed with TB
After developing the assays described in Table 1, at 25 °C the highest D% (99.6%) was obtained with the conditions of assay 6 (pH 7.05, 0.6 mM 2,5-dimethylaniline, 12.5 g L-1 glucose, 10.1 g L -1 ammonium tartrate and in the absence of copper sulfate). Similarly, at 30 °C a 99.4% of decolorization was achieved under the conditions of the assay 15 (pH 7.06, 1 mM 2,5-dimethylaniline, 12.5 g L-1 glucose, 10.1 g L-1 ammonium tartrate and 1.6 mM CuSO4 5H2O); while at 35 °C the highest decolorization (97.9%) was achieved with assay 20 (pH 3.04, 0.2 mM 2.5-dimethylaniline, 5 g L-1 glucose, 0.2 g L-1 ammonium tartrate and 0.2 mM CuSO4 5H2O). Overall, in most of the experiments carried out at 25 °C and 30 °C, high D% values were obtained, indicating that at these temperatures the decolorization process enhances; whereas, at 35 °C, except to assay 2 (D%= 95; pH 3.03, 0.2 mM 2.5-dimethylaniline, 20 g L-1 glucose, 0.2 g L-1 ammonium tartrate and 3 mM CuSO4 5H2O) and assay 20 (D% = 98), most of the experiments showed low D% or nulls (Fig. 2).
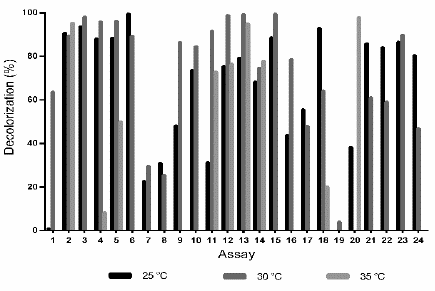
Source: The authors.
Figure 2 Decolorization percentage (D%) of wastewaters dyed with TB (200 mg L-1), by action of the fungus Leptosphaerulina sp. at 25 °C, 30 °C and 35 °C, 200 rpm, t= 15 days.
Table 2 Experimental design to evaluate TB removal by Leptosphaerulina sp. at 28 °C, t= 15 days.
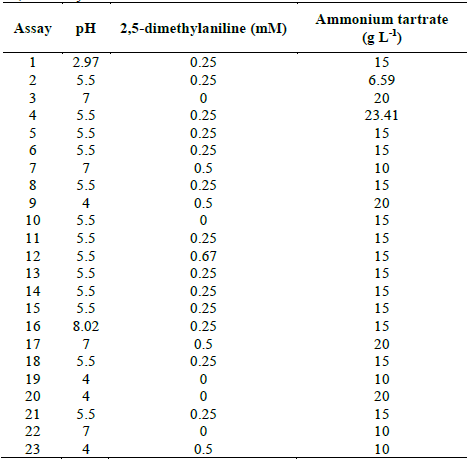
Source: The authors.
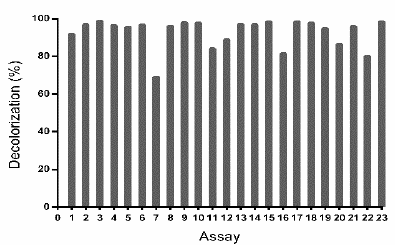
Source: The authors.
Figure 3 Decolorization percentages (D%) of the waters dyed with TB dye by Leptosphaerulina sp. Experimental conditions: 28 °C, 200 rpm, t= 15 days.
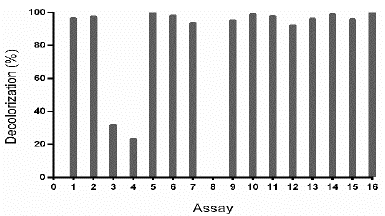
Source: The authors.
Figure 4 Decolorization percentage (D%) of TB by Leptosphaerulina sp., under conditions of the experimental design specified in Table 3. Experimental conditions: 28 °C, 200 rpm, t= 8 days.
Table 3 Central composite design for optimization of D% of TB by Leptosphaerulina sp., depending on pH and ammonium tartrate.
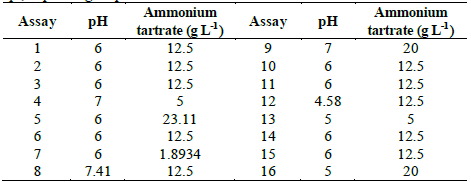
Source: The authors.
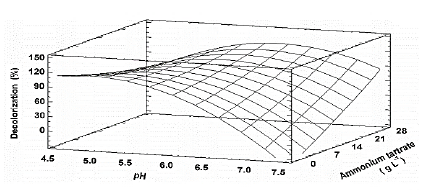
Source: The authors.
Figure 5 Estimated response surface for the decolorization percentage (D%) of TB, by Leptosphaerulina sp. (t= 8 days), depending on pH and ammonium tartrate.
The previous results led to the proposal of a second response surface experimental design, of 23 assays (Table 2), considering a fixed temperature at 28 °C, in-between those in which the experiments had better outcomes (25 °C and 30 °C). In addition, copper sulfate was excluded and the glucose concentration was fixed at 10 g L-1, owing to the fact that these compounds did not have a significant impact on the response variables obtained in the first experimental design (Table 1). When developing the experiments at 28 °C, decolorization percentages higher than 75% were obtained, with the exception of assay 7, which was near to 70% (Fig. 3).
In Table S1 is observed that ammonium tartrate and the interaction between pH and ammonium tartrate had a significant effect on TB removal at 28 °C. Similarly, variance analysis results suggest that the optimization of the process does not require 2,5-dimethylalanine (p-value > 0.05). For that reason, the effect of the same factors was evaluated, through a central composite design that included pH values in the range 4.58 - 7.41 and ammonium tartrate between 1.89 and 23.11 g L-1, giving rise to 16 assays (Table 3).
Table S2 shows the significance of the effects that make up the model adjusted for the design specified in Table 3. Both, the lineal and the quadratic effect of the pH on the D% are significant (p-value < 0.05). Likewise, ammonium tartrate and its interaction with the pH have a significant effect on TB removal. Fig. 4 shows the results obtained for the assays under the conditions specified by Table 3. The adjusted model explained 92.79% of the variability in the TB decolorization, at day 8. The optimization indicates that decolorization was maximized when the process was conducted at pH 6 and with a concentration of ammonium tartrate of 23.11 g L-1 (Fig. 5). The regression equation that fitted to the models for D% at day 8 is shown below (eq. (2)):
Where X1 is the pH y X2 is ammonium tartrate concentration.
To corroborate the above-described results, another central composite design was done, exploring a region around the point obtained as optimum (pH 6 and ammonium tartrate 23 g L-1) (Table 4). As presented in Fig. 6, to all assays except the assay 5, TB removal was ~ 100%.
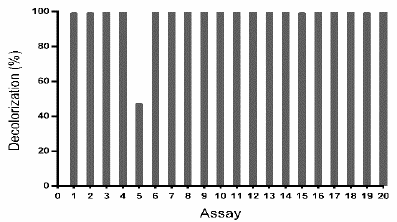
Source: The authors.
Figure 6 TB removal (%) by Leptosphaerulina sp. (t= 8 days), under the experimental design specified in Table 4.
Considering that the design explores a narrow region around the optimum, the adjusted model was approximated as a horizontal plane; consistently, significant effects of the studied variables were not observed. This signifies that a decolorization ~100% was expected for any combination of the factors in the evaluated region, which is coherent with results in Fig. 6.
3.2 Enzymatic activities
In order to know the relationship between the enzymatic activity of Leptosphaerulina sp. fungus and its capacity to remove the TB dye in aqueous solution, the enzymatic activities Lac, MnP and LiP were determined for all assays of the experimental designs registered in Table 1, 2 and 3. In Fig. S1 Lac activity was presented. MnP activity was low in all evaluated cultures (data not shown) and LiP activity was not registered in any experiment.
When the design of Table 1, at 25 °C, was analyzed for enzymatic activities, the assays 3, 13, and 22 reached the highest Lac activity with the latter having 168 U L-1 (Fig. S1) while at 30 and 35 °C the predominant Lac activities only reached values of 77.5 UL-1 (assay 18) and 5.7 U L-1 (assay 4), respectively.
In the experiments at 28 °C (Table 2), the predominant Lac activity (129 U L-1), was obtained with the combination of factors of the assay 5 (Fig. S2). Such activity was superior to that found by Babic et al. (2012) using a similar statistical methodology, who reported 41 U L-1 in a bioremediation study [47]. In addition, in assay 8, 14.43 U L-1 of MnP activity was measured (results not shown). Novotny, et al., (2004), used a similar methodology based on response surfaces and reported a maximum Lac activity of 46 U L-1 and MnP activity of 204 U L-1 [48].
In the confirmatory experimental design (Table 4), the maximum value of Lac enzymatic activity (197.068 U L-1) was reached with the condition of assay 1 (Fig. S3); however, a statistically significant relationship between enzymatic activity and the input variables in this design (pH and ammonium tartrate) was not observed.
As observed in Fig. S4, there was not a relationship between D% and the Lac activity. In the evaluated region, for all assays D% ~ 100% were obtained, without a correlation with the enzymatic activity. This result suggests that the TB was removed by a mechanism different from the enzymatic. This result coincided with the qualitative evaluation of TB removal by Leptosphaerulina sp. As it can be seen in Fig. S5 the fungus grows in pellet shape, which was clearly dyed with TB, indicating that biomass sorbed the dye. Confronting the quantitative and qualitative results, it can be estimated that under the operating conditions of this research, Leptosphaerulina sp. removed the dye mainly by the biosorption mechanism, with a good performance.
3.3 Quantification of dyestuff TB sorbed by Leptosphaerulina sp. biomass
As previously stated, dyes can be decolorized by degradation through enzymatic machinery of the fungi or by biosorption into the cell wall; to distinguish between these two possibilities, we evaluated the desorption of TB using different solvents and sonication but the dye was strongly joined to the biomass and it was not possible to recover the dye in this way. Therefore, this was not a suitable methodology to quantify adsorption capacity by fungal biomass of Leptosphaerulina sp. even though this has been successfully employed with residual materials of plants [46]. As a result, another methodology was proposed, which consisted of the utilization of inactive biomass of Leptosphaerulina sp. in contact with TB and gently agitated during 8 days. By using this procedure was estimated that the TB decolorization by Leptosphaerulina sp. was carried out mainly by sorption mechanism (D% of 89%).
4 Discussion
Development of this research conducts to an optimum region for TB removal in aqueous solution by Leptosphaerulina sp., characterized by a culture media with pH between 5.5 and 6.5 and a source of nitrogen (ammonium tartrate), with concentrations of 21 g L-1 to 25 g L-1. In such region, ~100% of decolorization of dyed wastewater was reached; given that a direct relationship between the enzymatic activity and dye removal was not observed, we estimated that 89% that was decolorized corresponds to sorption phenomenon of the dye over the cell wall of the fungus and the remaining (10%) to oxidation of dye due to the effect of ligninolytic enzymes of the fungus, mainly Lac. Although this arose from the experiments of the central composite design (Table 3, Fig. S4), only until developing the biosorption experiment of TB dye on the biomass of Leptosphaerulina sp., the combined effect of sorption-enzymatic oxidation could be established with greater certainty, with superior participation of the biosorption phenomenon. This combined activity of sorption-oxidation was also reported by Plácido et al., (2016), who determined D% of dyed wastewater with Novacron Red and Remazol Black, using Leptosphaerulina sp. reaching 90% of decolorization by sorption and 5% due to enzymatic activity [37].
In terms of D% (~100%), the results found in this research are comparable with those obtained by Kirby et al., (1997), who prepared a synthetic matrix with 8 dyes and used the Phanerochaete chrysosporium and Coriolus versicolor fungi achieving 99% and 98% of decolorization, respectively [49].
This finding is also consistent with the report of Senthilkumar et al. (2014), who achieved a D% of 98% of Amido black 10B dye by using Phanerochaete chrysosporium. These authors noted a correlation between high decolorization and high enzymatic activity [50]. Kumari and Naraian (2016), reported 98% of brilliant green decolorization with the co-culture of Pleurotus florida and Rhizoctonia solani. In addition, Lac and MnP enzyme extract of these two strains showed the capacity to degrade the dye [50,51]; however, our outcome differs of the previously cited studies because they noticed a greater reduction of color with maximum levels of ligninolytic enzymes, such as MnP and Lac, whose production was stimulated by the presence of dyes while in our study, there was not a correlation between enzymatic activity and D% by Leptosphaerulina sp. the native Colombian fungus.
The statistical analysis showed a great influence of pH over the TB sorption, which has an anionic chemical nature. In all the experiments made, the pH range was in the acidic region of the scale (5.5-6.5) reaching D% higher than 90% in all cases. This finding is consistent with that of Taçtan et al., (2012), who identified an optimum pH 6 for the bioremotion of Maxilon Red GRL, Everdirect Fast Black and Brillant Blue R by the action of Aspergillus versicolor fungus. Particularly, with the Everdirect Fast Black, they observed the fastest uptake and a decolorization of 100% [52]. These results are also in agreement with those obtained by Corso et al., (2012), which found that decolorization of dyed wastewater with Direct Red 23 and Direct Violet 51, is given by sorption mechanism on Aspergillus oryzae pellets and that proceeds better at a pH 2.5 [28]. Similarly, Aksu & Çagatay (2006), found that pH is one of the most influential variables in Gemazol Turquise Blue-G adsoprtion on the cell wall of Rhizopus arrhizus, with a maximum adsorption at pH 2.0 [53]. Likewise, the biomass of Aspergillus niger showed the capacity to adsorb the azo dye Procion Red MX-5B at pH 4.0 reducing the color up to 30% and significantly decreasing the toxicity of the solution [54]. Thus, the above-cited authors coincide that low pH values favor removal of anionic dyes, such as phthalocyanine dyes, by sorption mechanism, although we suggest that when using active biomass, it is needed that the value of this variable also allows a rapid microbial growth.
Sorption capacity of the microorganisms is attributed to heteropolysaccharides and lipids composing the cell wall, which contain in their chemical structure different functional groups such as amino, carboxyl, hydroxyl, phosphate, and other charged groups, which cause attractive forces between dyes, compounds of anionic structures, and the cell wall. Some authors [55-57] reported that the removal of anionic dyes in aqueous medium due to sorption phenomenon in fungal biomass is favored at acid pH, since the protonation of weak bases present in the cell wall is increased, acquiring a positive net charge, which subsequently joins with the anionic groups of the dye.
Nitrogen source was the other operational variable with a determinant role in TB bioremediation by Leptosphaerulina sp. These results match those observed in an earlier study by Kapdan et al. (2000), who achieved 96% of decolorization of the phthalocyanine dye Everzol Turquoise Blue in aqueous solution with the species Coriolus versicolor, in presence of ammonium tartrate, one of the most used salts as nitrogen source in fungal decolorization of dyes; nonetheless, the previously mentioned authors recommend to use urea as nitrogen source because is cheaper as well as to use a low concentration of nitrogen for an effective removal of color, an outcome which is contrary to the results of the present study [58]. Likewise, most recent studies have analyzed the role of nitrogen sources in dyes decolorization. Tehrani et al. (2014) have reported that the addition of ammonium chloride supported decolorization of pigment yellow (PY-74) and reactive blue (RB-38) using different fungal strains, although the concentration of this compound was significantly low (0.2 g L-1) compared with the used in the present study [59]. Nitrogen source showed a marked impact in D% of dye Navy blue HER (Reactive Blue 171) using the fungal strain Marasmius sp. BBKAV79 with the maximum decolorization being reached when peptone was the nitrogen source, while there was no decolorization in the presence of urea and ammonium chloride which differs from the findings presented here [60].
In addition, it was observed that when performing the bioprocess to decolor waters stained with TB (200 mg L-1) with Leptosphaerulina sp. and applying the optimal values for the significant variables, ammonium tartrate and pH, 99% is achieved of decolorization in two days of culture (data not shown). In accordance with the present results, previous studies have demonstrated that biosorption involves a faster removal of a large number of dyes, which facilitates the removal of the source of toxicity [28]. This study also supports our deduction, in that decolorization of the TB by Leptosphaerulina sp. fungus, gives priority to the mechanism of biosorption (D% 89) over the enzymatic (D% 10). In agreement with the present results, previous studies have demonstrated that this dual decolorization mechanism (biodegradationbiosorption) is present in many fungal species e.g. Aspergillus niger and Phanerochaete chrysosporium [61].
The experiments carried out indicate that Leptosphaerulina sp. the native fungus of Colombia could be used for the removal of phthalocyanine TB in vivo or as inactive biomass. In the first case, we propose the growth of the fungus in pellets because it favors the sorption as stated by other authors [28] since there is a larger superficial area, which is translated in a better enzymatic and physic contact with the surroundings [57]. As such, probably the decolorization at the present research was favored by Leptosphaerulina sp. growth in pellets (Fig. S5). The morphology of the biomass is an important feature of the culture because it affects decolorization process efficiency, as an example, aerobic granular sludge has a much higher conversion capacity of pollutants in wastewater due to the higher active biomass concentration which in turns has the ability to degrade or adsorb contaminant present in solution, consequently, aerobic granular sludge has been successfully employed as adsorbent material to capture dyes Acid Yellow 17, Yellow 2G and Reactive Brilliant Red K-2G from aqueous solutions [62]. Furthermore, the morphology attained in our experiments facilitates solids-liquid separation processes due to superior settling properties [63,64].
One possible advantage of inactive biomass is that by applying it, operating costs would be reduced, with which the potential for industrial application increases. For this purpose, packed-bed type reactors could be used, as Charumathi & Das (2012) did, by using non-living biomass of yeast Candida tropicalis, as an efficient bio-adsorbent, for the removal of synthetic dyes present in wastewater [65]. Moreover, the characteristics of the cell surface of the biomass (sorbent), in relation to the dye adsorption mechanisms, can be improved by subjecting it to heat, as in the present study. It is assumed that this treatment can lead to an increase in cell surface charges or openly available sites for adsorption and improves ion exchange [66]. Non-viable biomass of Aspergillus fumigatus showed an improved capacity to remove methylene blue from aqueous solution when treated by heat [67].
Finally, owing to the ability of Leptosphaerulina sp. to remove TB dye from aqueous solution and the numerous studies reporting biosorption capabilities of different fungal strains to uptake diverse kinds of pollutants, such as dyes [68] and heavy metals [69], future studies for biomass characterization of Leptosphaerulina sp. are suggested to know adsorption capabilities and adsorption kinetics of this low cost and easy-to-obtain material.
5 Conclusions
The decolorization process of water doped with the TB synthetic dye, using Leptosphaerulina sp. the native fungus achieved near 99% removal, based mainly on the removal of the dye by sorption mechanism (89%). Although ligninolytic enzymatic activity (10%) is present, there is no direct relationship between the enzymatic activity and the removal of the dye. This allows considering the potential of the bioprocess, for applications in real matrices dyed with this type of dyes. The response surfaces experimental design based on a central composite design, allowed determining the optimal conditions (pH and ammonium tartrate concentration) to decolorize TB. The pH showed to be a fundamental variable in the decolorization process of waters dyed with TB, favoring the biosorption phenomenon. The biomass of Leptosphaerulina sp., in the range of pH 5.5-6.5, is an excellent adsorbent for the removal of dyes in water.