Introduction
Wet brewer’s grains are an agroindustry residue generated during the brewing process and represents approximately 85% of the total residue obtained in this process (Mussatto et al., 2006). The high protein content of brewer’s grains makes this ingredient an alternative protein source for ruminants and can replace traditional feedstuffs such as soybean meal, with several advantages: lower cost, does not compete with human nutrition, and contributes to sustainability of animal production (Negesse et al., 2009).
However, the high moisture content of this residue is the most limiting factor in its use because it makes it difficult to transport, store, and preserve, making it more susceptible to development of molds, yeasts, and deterioration (Wang et al., 2014). Thus, dehydration is an alternative for the preservation of brewer’s grains. Dehydration is interesting because, in addition to avoiding quality loss, it decreases the volume of the material facilitating storage. However, dehydration using high temperature is not economically viable. Moreover, even when the dehydration temperature used is less than 60 °C there is a risk of toasting the grains (Mussatto et al., 2006) and reducing its nutritional value due to complexation of proteins with cell wall compounds, rendering them unavailable (Pereira et al., 1998). Alternatively, sun dehydration offers advantages, such as cost reduction and drying at lower temperatures.
After dehydration, chemical and physical modifications of the residue during storage are possible, especially if the storage site lacks adequate ventilation and protection against moisture. In addition, storage time itself may interfere with quality due to the aging of the product. Therefore, monitoring waste quality is necessary to determine the ideal storage period, thus ensuring that it can be used safely in animal feed.
In this context, the objective of this study was to evaluate the efficiency of dehydration of wet brewer’s grains (WBG) in the sun, as well as the effect of storage period on the nutritional and microbiological quality of the dried byproduct (DBG).
Materials and Methods
Ethical considerations
The experimental protocol for the use of cannulated ruminants was approved in 2013 by the Unioeste Animal Use Ethics Committee (Protocol 35/13).
Location
This study was divided into two experiments conducted at Universidade Estadual do Oeste do Paraná (Unioeste), Marechal Cândido Rondon - Brazil. In the first experiment, the wet dehydration process of WBG was evaluated. The second study investigated the influence of storage period on the nutritional and microbiological quality of the DBG.
Experiment 1
A total of 6,000 kg WDG was acquired from a brewery located in Toledo, Paraná- Brazil, with 206 g/kg dry matter (DM) content. The drying of the residue was conducted under the sun by uniformly distributing it on a concrete floor, forming a layer of approximately 1.5 cm, with a density of 0.998 kg of dry matter/m². The material was scattered and turned every hour. The WBG remained dispersed for dehydration for two-day and one-night period periods, totaling 31 hours. The residue remained exposed to the sun for 16 hours, and only those hours were considered for the dehydration rate due to the presence of sunlight. The period of sunlight exposure ranged from the moment that sun's rays began to directly fall on the brewer's grains until sunlight ended in the evening. At night, the residue was covered with a black tarpaulin to prevent reabsorption of moisture.
A completely randomized experimental design was used to determine dehydration. The treatments consisted of collection times during drying, with 10 repetitions. The sampling times were: WBG was considered as time 0 and 1, 2, 4, 6, 8, 10, 12, 14, and 16 hours of dehydration, with the schedule of 9:00, 10:00, 11:00, 13:00, and 15:00 on the first day of drying, and at 8:00, 10:00, 12:00, 14:00, and 16:00 on the second day of drying. The particle size of the collected material was from 3 to 8 mm. The material was placed in paper bags and pre-dried in an oven at 55 °C for 72 hours to allow subsequent grinding. Afterwards, it was ground in a knife mill (Tecnal model TE 650/1, Tecnal Laboratory Equipment, Piracicaba, SP, Brazil) and analyzed for DM content according to AOAC (1990; method 934.01). During sampling, temperature, relative air humidity, solar radiation, and wind speed during the drying days were obtained from the Meteorological Station of Marechal Cândido Rondon, Paraná, Brazil.
Statistical analysis
Normality of the variables was verified using the Shapiro-Wilk test. Dehydration data were analyzed by PROC REG of SAS software, version 9.0 (SAS Institute Inc., Cary, NC, USA; 2002) testing linear and quadratic models. Significance was declared as p<0.05.
Experiment 2
After dehydration under the sun, a second test was carried out with the purpose of evaluating possible changes caused by storage time on the DBG product. For this, a completely randomized design with nine storage times was used.
After dehydration, the DBG was collected, packed in polypropylene braided bags (Copagril, Marechal Cândido Rondon, PR, BR) and stored in a 15 x 20 m covered shed. Residue samples collected after dehydration were considered as 0 storage day. Four samples (repetitions) were collected for 10, 20, 30, 60, 90, 120, 150, and 180 days of DBG storage. Each sample was composed of 5 subsamples collected from different parts in the bag. The samples were ground in a 1 mm sieve (Star FT-80/2, Fortinox, Piracicaba, SP, BR) and analyzed for DM, ash, crude protein (CP), and ethereal extract (EE) according to AOAC (1990) by the methods 934.01, 938.08, 981.10, and 920.85, respectively. Neutral detergent fiber was analyzed according to Van Soest et al. (1991) and adapted for the Ankom 2000 Fiber Analyzer (Ankom Technology, Macedon, NY, USA) and later corrected for ash and protein (NDFap). Total carbohydrates (TC), non- fibrous carbohydrates (NFC), and carbohydrate fractionation were calculated according to the equations proposed by Sniffen et al. (1992). Protein fractionation was performed according to Licitra et al. (1996).
Animals, location, and in vitro procedures
For in vitro procedures, ruminal fluid was collected from three cannulated Jersey steers from the Unioeste Experimental Farm. The animals were kept in Tifton grass picket (Cynodon sp.) receiving feed in the corn silage trough and concentrate composed of ground corn, soybean meal, DBG, and mineral mix.
In vitro gas production was obtained using the methodology of Theodorou et al. (1994) and modified by Mauricio et al. (1999) in a computerized wireless system (Ankom RF Gas Production System, Ankom Technology, Macedon, NY, USA). For this, 500 mg of sample was introduced into a glass flask (295 mL), along with 100 ml buffer solution (pH 6.8) and 25 mL rumen fluid. For the variation adjustments, flasks considered blank were incubated, containing the incubation solutions without substrate. Gas pressure produced in the flask was measured every 10 minutes for 48 hours. Accumulated gas volume was corrected for the fermented DM. To estimate ruminal fermentation kinetic parameters of each treatment, the bicompartmental logistic model was adjusted to the cumulative gas production curves as proposed by Schofield et al. (1994).
In vitro dry matter digestibility (IVDMD) was estimated by the technique described by Tilley and Terry (1963) and adapted by Holden (1999), using incubator model TE-150 Tecnal (Tecnal Laboratory Equipment, Piracicaba, SP, Brazil). In vitro crude protein digestibility (IVCPD) was determined by the residual protein analysis after incubation. The technique of Goering and Van Soest (1970) was used for the determination of in vitro cell wall digestibility (IVCWD).
Microbiological analysis
For fungi analyses, 25 g of sample was added in 225 mL sterile distilled water and maintained with continuous stirring for five seconds. From this solution 1 mL was pipetted in successive dilutions of 10-1 to 10-5 using test tubes containing 9 mL distilled water. Subsequently, from the diluted extracts, surface seeding was performed on the plates using 0.1 mL inoculum per plate. The inoculum was spread on the plate with the aid of a Drigalski loop.
Potato dextrose agar (PDA, Acumedia, Lansing, MI, USA) was used as culture medium at pH 3.5 and acidified with tartaric acid (10:100 w/v; Synth, Diadema, SP, BR). The plates were incubated at 30 °C for 3-5 days. Subsequently, the fungal colonies were counted being considered only plaques with less than 100 colonies (APHA, 1992). Identification of the genus was performed according to Samson et al. (1996) through the observation of microscopic characteristics of the spores in each colony. Microscope slides were prepared by the scraping method, using cotton-blue as dye. Total aflatoxins was determined in a fluorimeter (Vican Series-4X fluorimeter, Vican, Miliford, MA, USA) using the AflaTest® methodology (AflaTest Micotoxin Testing System, Vican, Miliford, MA, USA).
Statistical analysis
Normality of variables was verified using the Shapiro-Wilk test. The data of fungi genus population were transformed into Log base 10. Data were analyzed by PROC REG of SAS software, version 9.0 (SAS Institute Inc., Cary, NC, USA; 2002), testing linear and quadratic models. Significance was declared as p<0.05.
Results
Experiment 1
Average ambient temperature and relative humidity during WBG dehydration were 25.6 °C and 64.2%, respectively (Table 1). Dry matter content of WBG increased linearly (p<0.05) with dehydration (Figure 1).
Experiment 2
The DM content of DBG presented changes (p<0.05) during storage (Figure 2). Contents of ash, CP, EE, NDFap, and NFC had no effect (p>0.05) as a function of the storage times tested (Figure 3).
Table 1 Temperature of wet brewer’s grains (TWBG, °C), ambient temperature (AT, °C), relative humidity (RH, %), solar radiation (SR, kJ/m2) and wind speed (WV, m/s) during the dehydration process of wet brewer’s grains.
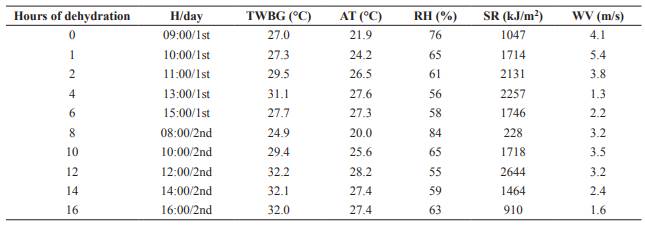
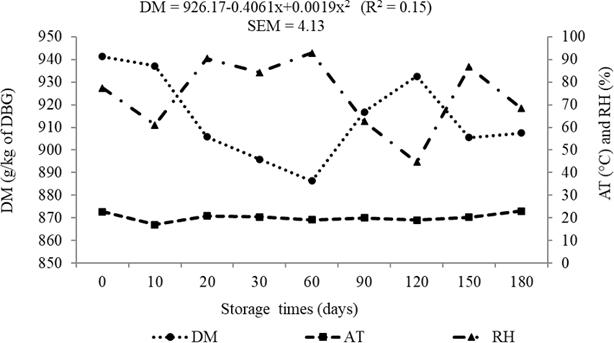
Figure 2 Dry matter (DM) of dried brewer’s grains (DBG), ambient temperature (AT) and relative humidity (RH) during storage period of DBG.
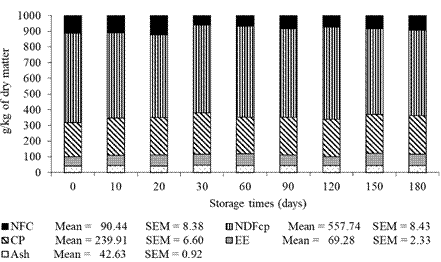
Figure 3 Ash content, crude protein (CP), ether extract (EE), neutral detergent fiber corrected for ash and protein (NDFap), and non-fibrous carbohydrate (NFC) of dried brewer’s grains during storage.
Storage days of DBG did not influence (p>0.05) fractions A, B1, B2 and B3of the protein (Table 2). Fraction C, considered indigestible,increased linearly (p<0.05) with storage time. Carbohydrate fractions A+B1, B2 and C had no effect with increasing storage time of DBG (p>0.05).
The gas volume of the fast degradation fraction (A) showed a quadratic effect as a function of storage time, with the maximum estimated at 88 days, while degradation rate of non-fibrous carbohydrates (B) showed linear increasing effect (p<0.05) (Table 3). Time of colonization (C), gas volume (D), and degradation rate of the slow fraction (E) as well as total gas production (A+D) had no effect as a function of storage time (p>0.05). Dried brewer’s grains storage time did not influence (p>0.05) IVDMD, IVCPD, and IVCWD.
Table 2 Fractionation of proteins (CP) and carbohydrates (TC) from dried brewer’s grains submitted to different storage times.
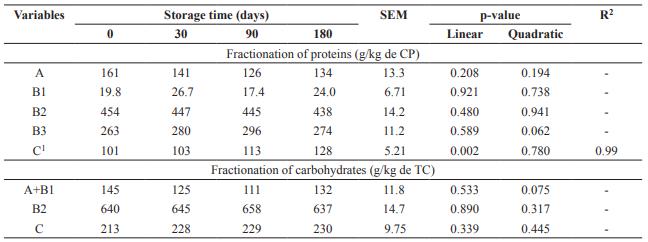
SEM: Standard error of the mean. Fractionation of proteins: A: soluble; B1: rapid ruminal degradation; B2: intermediate ruminal degradation; B3: slow ruminal degradation; C: indigestible. Fractionation of carbohydrates: A+B1: rapidly degradable; B2: potentially degradable fibrous carbohydrates; C: non-degradable carbohydrates. 1 Ŷ= 99.9755 + 0.1540x.
Storage time did not affect DBG fungal population (p>0.05). The most common genotypes of fungi found in all evaluation times were Cladosporium sp. and Fusarium sp.
(Figure 4a). Total aflatoxin concentration (Figure 4b) showed a quadratic effect as a function of storage time (p<0.05) with the maximum estimated at 96 days.
Table 3 In vitro cumulative gas production and in vitro digestibility of dried brewer’s grains submitted to different storage times.
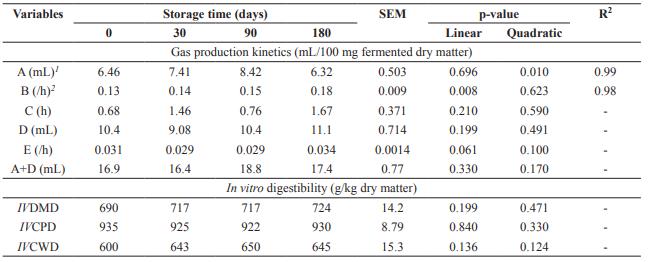
SEM: Standard error of the mean; A: gas volume from the fast degraded carbohydrate fraction (non-fibrous carbohydrate); B: rate of degradation of non-fibrous carbohydrate; C: latency or colonization time; D: gas volume from the slow-degraded carbohydrate fraction (fibrous carbohydrate); E: rate of degradation of fibrous carbohydrate; IVDMD: in vitro dry matter digestibility; IVCPD: in vitro crude protein digestibility; IVCWD: in vitro cell wall digestibility; 1 Ŷ= 6.4038 + 0.0442x - 0.00025x2; 2 Ŷ= 0.1337 + 0.00025x.
Discussion
Experiment 1
Average ambient temperature was 25.6 °C during dehydration, which may be considered low and suggests that wind speed, solar rays, and low relative air humidity also contributed to the drying of the material. Brewer’s grains may reduce protein availability during dehydration, as drying temperature increases due to condensation of some proteins with indigestible cell wall materials (Pereira et al., 1998). However, as it was dehydrated under the sun, the temperature was low so no change in composition was expected (Pereira et al., 1998).
Dehydration of WBG showed increasing linear effect on DM content of the material. The DM content increased 49.82 g/kg per hour of drying. With this dehydration rate WBG resulted in a DM matter content of 949.85 g/ kg at the end of the dehydration period. The DM obtained at the end of dehydration was sufficient to ensure the preservation of the residue, and exceeds the values obtained by Schone et al. (2016), who observed DM content around 880 g/kg when dehydrating brewer's grains in the sun in a 2 cm-thick layer. Industrial dehydration of WBG results in DM content between 900 g/kg (Aliyu and Bala, 2011) and 923 g/kg (Dihman et al., 2003).
Experiment 2
The DM content of DBG presented changes during storage. This behavior is associated to air humidity at each storage time. Sun dehydration was conducted during the month of April, so collection for the 10, 20, 30, and 60 storage days occurred when air humidity was high, causing reabsorption of atmospheric moisture by the residue. The reduction of air humidity that occurred in the following months was responsible for the new increase in DM content of the material.
Storage time did not influence the chemical composition of DBG; however, some oscillations observed in nutrient concentration were mostly due to the heterogeneity of the product, which is caused by grain variety, harvest time, maceration conditions and malting (Robertson et al., 2010), type of beer produced, cereals added together with barley such as corn, wheat and rice (Mussatto et al., 2006), production method, and waste recovery (Westendorf et al., 2014). Additionally, DBG does not have a defined quality standard for commercialization because it is an agroindustry residue.
Storage days did not influence carbohydrate fractionation of fractions A, B1, B2, and B3 of the protein, but fraction C increased with storage time. For each day of storage this fraction increased 0.16 g/kg of CP, so part of the previously digestible protein was bound to the cell wall compounds, becoming unavailable.
Gas volume of the fast degradation fraction (A) showed a quadratic effect as a function of storage time, while degradation rate of non- fibrous carbohydrates (B) showed a linear increasing effect. Although these variables were significant, the results may also have occurred due to heterogeneity of the product. Gas volume of the rapid degradation fraction was low for all storage times since soluble carbohydrates and barley starch are largely solubilized during the malting and brewing stage, generating low-grade brewer’s grains of fermentable carbohydrates.
Gas volume of the slow fraction (D), total gas production (A+D), and in vitro digestibility of DM, CP and cell wall had no effect as a function of storage time. High gas production of the slow degradation fraction occurred due to high concentration of fibrous carbohydrates, since DBG is mainly composed of barley, which is rich in cellulose, non-cellulosic polysaccharides, and lignin (Mussatto et al., 2006).
Fungal population of DBG was not altered with increasing storage time. The most common genotypes of fungi found in all evaluation times were Cladosporium spp. and Fusarium spp. Genus Rhizopus spp. was the lowest in all storage times, while genus Phoma spp. was found only after 30 days of storage. Keller et al. (2012) obtained larger populations of Cladosporium spp., Aspergillus spp. and Mucor spp., but also less frequently isolated fungi Eurotium spp., Penicillium spp., Alternaria spp., and Fusarium spp. Gonzalez-Pereyra et al. (2011) observed greater contamination by Fusarium spp. (30%), Mucor spp. (30%), and Aspergillus spp. (27.3%). Other genera, such as Penicillium spp., Cladosporium spp., Geotrichum spp., and Alternaria spp. were also isolated.
Among the main genera of fungi observed, it is noticeable that Fusarium and Cladosporium are field fungi, which attack cereals during grain development, causing damage before harvest (Pezzini et al., 2005; Hofer et al., 2016). In addition, genera Aspergillus and Penicillium, considered the main post-harvest fungi (Morcia et al., 2013), were not detected, allowing to infer that most fungal populations present in DBG comes from contamination within the field, and the storage conditions were favorable for the preservation of the product. This statement was corroborated by Simas et al. (2007), who also pointed out that Aspergillus and Penicillium develop under inadequate storage conditions. When evaluating wet brewer's grains storage, these authors observed the presence of Aspergillus spp. as the most frequent genus (42.5%), followed by Mucor spp. (32.5%), Rhizopus spp. (32.5%), Penicillium spp. (7.5%), and Fusarium spp. (2.5%).
Total aflatoxin concentration showed a quadratic effect as a function of storage time. Values ranged from 35 to 45.5 μg aflatoxin/ kg DBG, but remained within the maximum tolerance limits of 50 μg/kg for animal feed and concentrate (Brasil, 1988). Simas et al. (2007) analyzed 80 samples of brewer’s grains finding that 33.75% of the samples tested positive for aflatoxin, with values between 1 and 3 μg/ kg. Gonzalez-Pereyra et al. (2011) detected concentrations of 19 to 44.52 μg aflatoxin B1/kg in 18% of brewer’s grains samples tested, while Keller et al. (2012) detected concentrations between 10 and 47 μg aflatoxin B1/kg in 4% of the samples.
Aflatoxin is produced by the fungi Aspergillus sp., but this genus was not detected in the present study. Gonzalez Pereyra et al. (2011) pointed out that detection of mycotoxins in a given substrate implies contamination by toxigenic species that may or may not be present at sampling time. Therefore, it is believed that contamination by Aspergillus sp. can occur through the barley grains during the field phase or during malting (Morcia et al., 2013). During brewing, subsequent to malting, the mash is heated to 78 °C for several hours (Gupta et al. 2010), which may have caused the disappearance of Aspergillus sp., thus maintaining the mycotoxins it produces, since they are thermostable (Terzi et al., 2014). Therefore, the absence of Aspergillus spp. and the maintenance of aflatoxin concentration in DBG within the acceptable limits during the storage time allow us to affirm that the storage conditions were favorable for the preservation of the product.
In conclusion, dehydration of WBG for a total period of 31 hours, with 16 hours of direct exposure to the sun and a density of 0.998 kg DM/m², is efficient to preserve the material and makes its storage feasible. Storing DBG for 180 days does not compromise its nutritional and microbiological quality. More research is needed on the use of technologies that provide renewable and low-cost energy, such as solar radiation, for the dehydration of this by-product on a large scale, considering its potential long- term storage for use in ruminant feed.