INTRODUCTION
Antioxidants are substances that in minimal concentrations delay or cancel the oxidation of different organic substrates. In living beings, they are of vital importance for the protection against oxidative damage produced by reactive oxygen species (ROS) -also known as free radicals- and other degenerative alterations (Maleki et al. 2019). ROS are highly reactive molecules with an unpaired electron in the outermost orbital, which are constantly produced during the course of many reactions essential for aerobic life, such as electron transfer reactions in the mitochondrial respiratory chain and also in certain cytoplasmic reactions (Zhao et al. 2020). From the pathophysiological point of view, ROS are involved in numerous diseases and tissue injuries such as those that occur at the pulmonary, cardiac, gastrointestinal, blood, ocular, cerebral, and hepatic levels and those associated with aging processes. The excessive production ofreactive oxygen species, particularly hydroxyl radicals, can alter membrane lipids with the subsequent production of lipid peroxides. Free radical-induced lipid peroxidation is a phenomenon that occurs in different alterations such as ischemia-reperfusion of tissues, chronic inflammation, the toxicity of certain drugs, etc. To counteract the effects mentioned above, antioxidants participate at different levels within the oxidative sequence through one of the following mechanisms: 1. decreasing the oxygen concentration by interception of singlet oxygen; 2. preventing the initiation step, scavenging hydroxyl radicals; 3. by decomposition of lipid peroxides, catalyzed by metal ions; 4. decomposing primary products to components without radical function; 5. preventing the breaking of the substrate chains by hydrogen abstraction (Zeb 2020). Antioxidants also act as hydrogen and/or electron donors to radicals formed from unsaturated lipids, thus stabilizing the active molecule (He et al. 2017). Natural antioxidants include phenolic and polyphenolic compounds, chelators, enzymes, and antioxidant vitamins. Vegetables in particular provide a rich source of natural antioxidants including vitamins C, E, carotenoids, and phenolic compounds. These substances have a similar basic molecular structure in which there is at least one aromatic ring and one hydroxyl group, such as phenolic acids, flavonoids and isoflavonoids, hy-drolyzable tannins, lignans, coumarins, stilbenes, flavanones, and oligomeric proanthocyanidins. These molecules act by different mechanisms, conferring an effective defense system against free radical attacks in plants (Dzugan et al. 2018; Van Hung 2016). In turn, the presence of an-tioxidants of plant origin in food prevents, among other alterations, cardiovascular problems, cancer, cataracts, etc. (Li et al. 2015). Among the organs susceptible to oxidative damage, the liver constantly faces the effects of ROS. It represents the largest gland in the body and has a central place in the metabolism of proteins, fats, and carbohydrates, it actively participates in the neutralization of potentially toxic substances (it synthesizes, among other compounds, glutathione) (Trefts et al. 2017), vitamin storage (regulates vitamin E levels), etc. In the case of poultry production, for example, the great advances in the genetic selection of production animals (greater growth speed and feed efficiency) must have been associated with increases in hepatic metabolic activity (Lambrecht et al. 2020). Birds raised in intensive production systems are subject to stress, a situation that promotes the production offree radicals. Broilers suffering from pulmonary hypertension syndrome or ascitic syndrome result in significant economic losses in world poultry farming and the presence of oxidative damage or reduction in the antioxidant capacity associated with these and other diseases has been documented (Xu et al. 2021). That is why in intensive poultry farming substances with antioxidant capacity are used. The hepatoprotective effect of SM in poultry farming is poorly understood, although it may be used as a cheap, nontoxic, and safe feed additive to manage liver-related disorders and to replace synthetic drugs in poultry diets (Saeed et al. 2019). SM was initially found in the mountains of the Mediterranean, Asia, and North Africa regions; however, today, it is grown in many parts of the world. SM is a complex flavonoid isolated from the fruits and seeds of milk thistle (Silybum marianum) that contains a mixture of six flavonolignans (silybin, isosilybin, silychristin, isosilychristin, silydianin, and silimonin) and other flavonoids (taxifolin, quercetin, dihydrokaempferol, kaempferol, apigenin, naringin, eriodictyol, and chrysoeriol); among these, silybin is the principal active component (MacDonald-Ramos et al. 2021). Silibinin is used as an active ingredient in a wide spectrum of dietary supplements and has received much attention over the last decade as an herbal remedy (Rajnochová et al. 2016; Surai 2015). The objective of this work was to analyze the in vitro effect of SM on oxidative damage in mitochondrial and microsomal membranes of chicken liver. In order to know the benefits of plant antioxidants in broilers, different concentrations of SM (6.25; 12.5, and 25 µg/mL) obtained from a mother solution in sodium phosphate buffer (0.04 M, pH7.0) were used.
MATERIALS AND METHODS
Chemicals
SM was obtained from Sigma Chemical Co. (St. Louis, MO, USA). Bovine serum albumin (BSA) (Fraction V) was obtained from Wako Pure Chemical Industries Ltd, Japan. L (+) ascorbic acid, butylene hydroxytoluene (BHT), and phenyl-methyl-sulfoxide fluoride (PMSF) were obtained from Merck Laboratories. All other reagents and chemicals were analytical grade from Sigma.
Use of silymarin preparation
A stock solution was prepared with 10 mg of SM dissolved in 10 ml of distilled water. Different dilutions of the SM (6.25, 12.5, and 25 (µg/ml) obtained from the stock solution in sodium phosphate buffer (0.04 M, pH 7.0) were used.
Animals and preparation of mitochondria and microsomes
32-day-old Cobb chicks donated by Balanceados Patagónicos, Trelew, Argentina, were used for the study. Immediately after the arrival (one day of life) of the chicks at the experimental house, they were weighed (71.38 g). The birds were kept in boxes of 2 m2, which were maintained with balanced feed and water ad libitum. The animals were sacrificed by cervical dislocation; the livers were removed, cut into small pieces, and washed repeatedly with a 0.15 M NaCl solution. The homogenate was prepared by adding 3 ml per gram of tissue of solution A (0.25 M sucrose; 10 mM Tris HCl buffer pH 7.4, 0.1 mM PMSF) in a potter Elvehjem homogenizer. The homogenate was centrifuged at 1,000 x g, the pellets were discarded, and the resulting supernatant was centrifuged at 20,000 x g for 10 min to obtain mitochondria. After centrifugation, 5 ml of the resulting supernatant was passed through a Sepharose 4 B column (1.6cm x 12 cm) equilibrated and diluted with 10 mM Tris-HCl buffer pH7.4 0.01% Na N3. The microsomal fraction appeared in the void volume (12-20 ml) and the cytosol was discarded. All operations were performed at 4 °C and under dim light. The quality of this microsomal preparation is compositionally similar with respect to concentrations and activities of certain microsomal enzymes to that obtained by ultracentrifugation (Tangen et al. 1973). Mitochondria were obtained by the method described by Schneider and Hogeboom (1958).
The CICUAL (Institutional Committee for the Care and Use of Laboratory Animals) of the Faculty of Veterinary Sciences, UNLP, approved all the housing and experimentation procedures
Peroxidation of chicken liver mitochondria and microsomes
Chemiluminescence and peroxidation were initiated by adding ascorbate-Fe++ to mitochondria and microsomes (1 mg protein) (ascorbate-Fe++ group) (Wright et al. 1979). To carry out the experiments, three groups were established: 1. control group (only organelles), 2. ascorbate-Fe++ group (organelles + inducer), and 3. SM group (organelles + inducer + SM). SM added mitochondria and microsomes (6.25, 12.5, and 25 µg-SM group) were incubated at 37 °C with 0.01 M phosphate buffer pH 7.4, 0.4 mM ascorbate, final volume 2 ml. The phosphate buffer is contaminated with enough iron to provide the necessary ferrous or ferric iron (final concentration in the incubation mixture was 2.15 µM) for peroxidation (Tadolini and Hakim 1996). Simultaneously, preparations were made with mitochondria and microsomes, which lacked ascorbate (control group). Light emission from the membranes was determined over a period of 120 min, chemiluminescence was recorded as cpm every 10 min, and the sum of the total chemiluminescence was used to calculate cpm/mg protein. Chemiluminescence was measured as counts per minute on a Packard 1900 TR liquid scintillation analyzer with chemiluminescence software.
Protein determination
Proteins were determined by the method of Lowry et al. (1951), using BSA as a standard.
Statistical analysis
Data were expressed as means ± SD of thirty independent determinations. The statistical analysis used was the Student's t-test. The 0.05 level was selected as the point of least statistical significance. The statistical criterion of significance was selected at different p-values and was indicated in each case.
RESULTS
Light emission from chicken liver mitochondria during peroxidation
Incubation of chicken liver mitochondria in the presence ofascorbate-Fe++ resulted in peroxidation of the membranes as shown by light emission (chemiluminescence) when the control and ascorbate-Fe++ groups were compared. The values were 298.704 ± 9.72 in the control group, while 1617.68 ± 69.37 in the ascorbate-Fe++ group, showing a significance value of p < 0.0005. After incubation of mitochondria in an ascorbate-Fe++ system at 37 °C for 120 min in the presence of increasing amounts of SM (6.25, 12.5, and 25 of SM) per mg of protein, the values of cpm caused by light emission were lower (concentration-dependent) in the SM group than in the ascorbate-Fe++ group. The values were 1190.79 ± 104.19 with the addition of 6.25 (Jg SM/mg protein; 705.41 ± 152.44 with the addition of12.5 µg of SM/mg protein and finally 244.9 ± 29.05 cpm with the addition of 25 of SM/mg protein. The significance values were p < 0.005 with the 6.25 µg SM/mg protein dose, while a significance value of p < 0.0005 was obtained for the 12.5 and 25 µg SM/mg protein doses. Figure 1 shows the light emission of chicken liver mitochondria obtained from the control groups, ascorbate-Fe++ and the SM group.
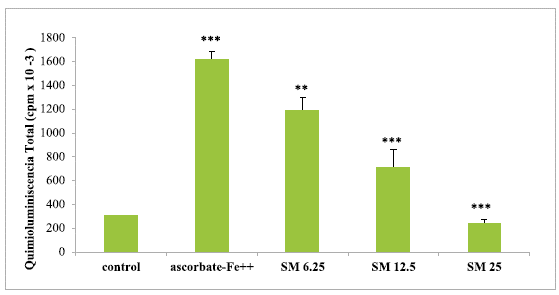
The results are expressed as the mean ± S.D. of thirty independent experiments. The light emission values show highly significant (**p <0.005) and highly significant (***p<0.0005) statistical differences between the compared groups.
Source: own elaboration.
FIGURE 1 Light emission of chicken liver mitochondria during peroxidation with different concentrations of SM.
Light emission from chicken liver microsomes during peroxidation
Incubation of chicken liver microsomes in the presence of ascorbate-Fe++ resulted in peroxidation of the membranes as evidenced by light emission (chemiluminescence) when the control and ascorbate-Fe++ groups were compared. The values were 347.58 ± 29.18 in the control group while in the ascorbate-Fe++ group, it was 1440.56 ± 13.53, showing a significance value of p < 0.0005. After incubation of mitochondria in an ascorbate-Fe++ system at 37 °C for 120 min in the presence of increasing amounts of SM (6.25, 12.5, and 25 μg of SM) per mg of protein, the values of cpm caused by light emission were lower (concentration-dependent) in the SM group than in the ascorbate-Fe++ group.
The values were 1034.81 ± 56.13 with the addition of 6.25 μg SM/mg protein; 810.59 ± 20.96 with the addition of12.5 μg SM/mg protein and finally 377.83 ± 41.91 cpm with the addition of 25 μg SM/mg protein. The significance values were p < 0.05 with the 6.25 dose, while for the 12.5 and 25 μg SM/mg protein doses a highly significant value corresponding to p < 0.0005 was obtained. Figure 2 shows the light emission of chicken liver microsomes obtained from the control groups, ascorbate-Fe++ and the SM group.
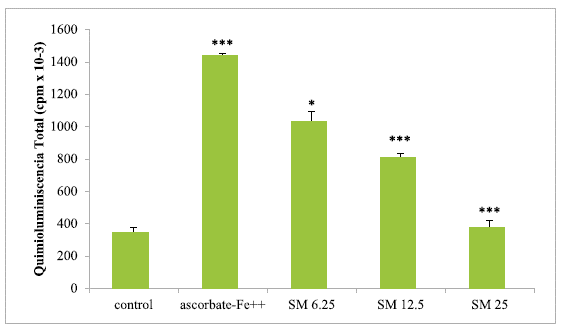
The results are expressed as the mean ± S.D. of thirty independent experiments. The light emission values show statistically significant (*p <0.05) and highly significant (***p<0.0005) differences between the compared groups.
Source: own elaboration.
FIGURE 2 Light emission of chicken liver microsomes during peroxidation with different concentrations of SM.
Comparative percentage inhibition of light emission in chicken liver mitochondria and microsomes
After incubation of mitochondria and microsomes in an ascorbate-Fe++ system(120 min at 37°C), it was observed that the percentage cpm/mg of protein from light emission (chemiluminescence) was lower in liver mitochondria and microsomes from chicken with the addition of SM. Thus, the highest percentage of inhibition of peroxidation observed with the addition of SM was 84.86% in mitochondria and 73.77% in microsomes for the dose of 25(µg of SM/mg of protein (figure 3).
DISCUSSION
During cellular respiration, univalent reduction of oxygen occurs (Oddone et al. 2019) and therefore most of the ROS formed under physiological conditions are different forms of oxygen radicals: superoxide anion, hydroxyls, and singlet oxygen. The balance between the generation of free radicals and their neutralization is controlled by complex mechanisms that include: a. the maintenance of the spatial separation between the sites of radical production and the biomolecules vulnerable to them, b. the enzymatic mechanisms of radical trapping: superoxide dismutase, catalase, and glutathione peroxidase, and c. the presence of substances with antioxidant properties: vitamin C, vitamin E, and glutathione (Parcheta et al. 2021). If the protective mechanisms are altered and the rate of ROS generation exceeds the antioxidant capacity, the effects produced by these reactive molecules can have great pathophysiological significance (Di Meo and Venditti 2020) on different cellular components: proteins, nucleic acids, and lipids having studied with greater emphasis the attack of ROS caused on lipids, an effect that leads to lipoperoxidation: the oxidation of the α-methylene bonds of unsaturated fatty acids that results in the formation of lipoperoxides and hydroperoxides, and finally the fragmentation of the lipid molecule (Zhong et al. 2019). Due to the fact that biomembranes are rich in polyunsaturated fatty acids, these reactions lead to the disintegration of the membrane structures and ultimately irreversible cell damage (Lawrence 2021). ROS have also been associated with cellular aging: the theory of progressive membrane aging emphasizes the damage produced to membrane fatty acids in the following aspects: a. membrane fatty acids are found in the primary site ofproduction of ROS (mitochondrial membrane) and are so close to them that no antioxidant defense system is capable of preventing their peroxidation; b. lipid peroxidation constitutes an autocatalytic chain reaction that, once started, will continue until it is eventually stopped by antioxidant mechanisms; c. many of the products of lipid peroxidation are themselves very reactive molecules and are therefore highly damaging agents on other molecules (Su et al. 2019). The aim of our study was to evaluate the protective in vitro effect of SM on chicken liver mitochondria and microsomes against peroxidation. In vitro peroxidation studies are useful to elucidate the possible mechanism of peroxide formation in vivo (Rauchová et al. 1993). Silymarin is used in humans in different liver disorders, particularly in chronic liver diseases, cirrhosis, and hepatocarcinoma, due to its antioxidant, anti-inflammatory, and antifibrotic power (Federico et al. 2017). Although considerable research has already been done to characterize changes in the structure, composition, and physical properties of membranes subjected to oxidation (Detaille et al. 2019; Gaschler and Stockwell 2017), it is important to know how biological compounds with properties antioxidants such as SM contribute to the protection of specialized membranes against the harmful effects produced by reactive oxygen species and other free radicals in other species, such as broilers in this case (Armanini et al. 2021). In the present study, we have determined that both chicken liver mitochondria and microsomes with the addition of SM were protected against peroxidation compared to similar membranes obtained from the ascorbate-Fe++ group, as shown by the results obtained by chemiluminescence. Our results are in agreement with previous work carried out with SM in rat kidney mitochondria and microsomes (Marmunti et al. 2016). Likewise, with the addition of the maximum dose of SM tested 25 µg/mg of protein, a higher percentage of peroxidation inhibition was observed in mitochondria than in microsomes in chicken liver. On the other hand, Saeed (2019) considers that the hepatoprotective effect of silymarin in poultry farming is poorly understood, but according to other authors it could provide a safe and cheap feed additive to promote growth performance and support liver function.
CONCLUSION
Our results are consistent with the hypothesis that SM can act as an antioxidant in cell membranes, showing greater protection in chicken liver mitochondria. However, further studies are needed to better evaluate these observations, in order to find an effective antioxidant dose in poultry.