Introduction
Population growth and urbanization are two of the predominant trends and challenges of the 21st century, since about 250 million people are added daily to the 7.2 billion who already live in Earth (Pimentel & Burgess 2018). The latter supposes an important pressure on ecosystems in order to guarantee the supply of food, water, fibers, energy, etc. to a population mainly concentrated in urban areas. Particularly, water supply is one of the main challenges to urban areas; e.g., large cities obtain 78 ± 3 % of their water from surface sources, some of which are far away: cumulatively, large cities move 504 billion liters a day (184 km3 yr -1 ) a distance of 27 000 ± 3 800 km (McDonald etal., 2014). However, as urban populations increase, total water supply must also grow to meet urban demand (Clercq et al., 2018). The latter places a great stress on urban water supply networks to find new sources and technologies; e.g., for seawater desalination (Topaloglu et al., 2018), Fog-water collection (Fessehaye et al., 2014) and groundwater treatment (Alvarez-Bastidaetal. 2018).
The treatment for drinking water involves the removal of mainly inorganic contaminants (suspended solids, iron (Fe), manganese (Mn), etc.) and undesirable components such as bacteria, viruses, fungi, etc, or reducing their concentration so that the water becomes safe for human consumption (Marsidi etal., 2018). These contaminants are accumulated in the different treatment units (decanters, filters, aeration systems, etc.) and then can be released into the environment by their maintenance and washing processes (Saleh, 2017; Saleh & Gupta, 2016).
Particularly, the concentration of metals such as Fe and Mn is high in wastewaters from drinking water treatment plants due to the pollution of superficial sources by landfill leachate sewage, chemicals from urban areas, agricultural or industrial activities such as mining or tanneries, and due to the pollution or composition of groundwater sources depending on the geology (Marsidi et al., 2018). The Fe is a transition element that is characterized by its ease in changing to the oxidation state [Fe (III)] and by its ability to form octahedral complexes with different bonds; it has a large variation of the redox potential of Fe (II) to Fe (III) (Juárez et al., 2007). Fe exists in two forms, which are soluble ferrous iron [Fe (II)] and insoluble ferric particulate iron [Fe (III); in water, the ferrous state is generally present (Marsidi et al., 2018). The Mn is a transition metal which is hard, brittle, refractory and easy to oxidize; it is found abundantly in the earth's crust, it has five oxidation states Mn (II), Mn (III), Mn (IV), Mn (VI) and Mn (VII) (Ipinza et al., 2007). Above specific levels, Fe and Mn can pose an unfavorable impact to both the environment and human beings (Marsidi et al., 2018), and are usually difficult to remove from water treatment systems; and sometimes the wastewater and solid waste generated in this process are also difficult to dispose of.
The discharges of liquids and solids with high metal concentrations of iron and manganese in the environment not only cause acute toxicity to aquatic organisms andplants, but also greatly reduce microbial and biological activity, as well as biodiversity and generate metallic sediments (Porter & Nairn 2010; Üstün, 2009; DeNicola & Stapelton, 2002), and when the pH is acidic, the water becomes strongly corrosive, altering its environmental conditions to the point of being unable to maintain various forms of life. Nevertheless, the wastewater generated by the maintenance of water treatment plants is generally discharged through the sewers or channels to the environment without any treatment, generating multiple impacts for the superficial or groundwater resources (Martínez, 2010; Gallego & Herráez, 2000). However, in many parts of the world such as Colombia, in the last years, the legislation obliges drinking water treatment plants to improve the quality of the water discharged by the maintenance of the treatment units.
To reduce and control water pollution by metals such as iron and manganese, many treatment systems have been developed; e.g., physical-chemical such as aeration, reverse osmosis or ion exchange; chemical such as neutralization and oxidation; and, biological treatments such as anoxic limestone drains, limestone open channels, bifurcated wells, biosorption, reactive mixing beds, successive systems of alkalinity production and wetlands, among others (Rios et al., 2008; Robbins et al., 1999; Moodley et al., 2018). Despite the development of all these treatment systems to reduce the negative environmental impact of wastewater with high concentration of Fe and Mn, important challenges still exist to adjust those treatments to changes in the environmental conditions and the physicochemical and biological characteristics of wastewater with metal contents (Diaz et al., 2003), as well as the development of treatments at reasonable costs to be adopted by the water treatment plants, principally in developing countries.
An advantage of biological treatments such as wetlands is that they use chemical substances of natural origin and biological processes for pollutants removal and with relatively low costs (Collins et al., 2005); they are simple to operate as they do not require skilled labor and construction costs are accessible to low resources communities. The operating costs of upward flow gravel filters to remove Fe and Mn indicate that they may result lower than that of a conventional physicochemical process (Marsidi et al., 2018). Also, a vertical superficial wetland can withstand total Fe and Mn concentrations up to 119 mg/L and 19 mg/L, respectively (Lopez et al., 2002), and requires little space for assembly.
The mechanisms of metal removal in wetlands are: i) Adsorption of the fine particles of the substrate and organic matter; ii) Precipitation as insoluble salts; ii) Induced absorption and change in the biogeochemical cycles of plants and bacteria; and iv) Precipitation as suspended solids due to low flow velocities (Marchand et al., 2010). Likewise, wetlands have mechanisms for the retention of Fe and Mn: i) Formation and precipitation of hydroxides; ii) Reduction by microbial action; iii) Complex organic reactions; iv) Exchange of cations with negatively charged materials; and v) Direct absorption by living plants (Knox et al., 2006). The latter depends on: i) The movement of metals from the environment to the root of the plant; ii) The transport of the metals towards the roots by the cortical membranes; iii) Transport of metals from the cortical cells to the xylem and from there to the root and stem; and iv) Mobilization of metals from leaves to storage tissues (seeds, fruits, tubers) through the phloem (Jeevanantham et al., 2019). In this context, the objective of this paper was to evaluate the effectiveness of vertical subsurface wetlands for Fe and Mn removal from wastewater from drinking water treatment plants, taking a pilot scale wetland with an ascending gravel bed with two types of plants: Phragmites australis (Cav.) Trin. ex Steudel and Colocasia esculenta (L.) Schott in El Hormiguero (Cali, Colombia), as an example.
Materials and methods
The vertical subsurface wetlands at a pilot scale was located in Cascajal at Hormiguero Township of Santiago de Cali (Colombia) for the treatment of wastewater of a drinking water treatment system by filtration under pressure. During 1976-2005 in the zone, the mean precipitation and the mean temperature oscillated between 1 036 mm and 1 427 mm and 22.1 ◦ C and 24 °C, respectively (CVC et al., 2017). The treatment system is supplied with groundwater and has two treatment lines with their respective oxygen injection system with a compressor; Gravel clarifier filter or upward pebble flow; fast flowing downstream sand filter; Ferric chloride dosing pump; and contact tank of ferric chloride. Within the operation and maintenance processes for each treatment line, the filtering material is washed weekly to ensure its continuous operation. 70 m3 of wastewater that has a reddish-brown color is discharged, with a maximum content of 216 mg/L of total suspended solids, 240 UNT of turbidity, 3.6 mg/L of total Fe and 2.6 mg/L of Mn.
The pilot plant had the following components: A tank of 1 000 L, a covered horizontal flow sedimenter, a covered flow distributor and three gravel filter of ascending flow where two types of vegetal species C. esculenta and P. australis were planted. Two gravel filters were planted with different species and the third one was left without a plant, acting as reference system of the experiment (Fig. 1). The pilot system was fed considering a hydraulic application rate of 1.2 m3 /m2 day, obtaining a value of 0.3 m3/day in each wetland and a filtration rate of 1.7 m/day. For the three wetland lines a total volume of 0.92 m3 per day was required; for this reason, a storage and homogenization tank of 1 m3 was used (Table 1). The flow of the wetland was ascending to make it easier for Fe and Mn hydroxides to be removed at the bottom of the filter, in addition to reducing the blockage of wetlands because if they are available in descending flow the iron can adhere to the rhizosphere of the plants. Also, C. esculenta and P. australis were chosen because macrophytes often diffuse oxygen in the surrounding substrate, creating oxidizing metal zones (Collins et al., 2004). Particularly, P australis is a cosmopolitan plant with root developments above 0.6 m (Rodriguez, 2003; Collins et al., 2005) and C. esculenta is a native plant which is well adapted to flooded media that can withstand the conditions used in this study, and also because this type of plant has also been used for the removal of mercury (Skinner et al., 2007).
The pilot plant was installed and was once it was stabilized the process of evaluation and monitoring of different parameters was started, such as pH, turbidity, concentration of Fe and Mn, among others; therefore, samplings were made from day 65 to the 171 day during March and June 2014. The samples were made at 14 points in the storage tank, flow control camera, sedimentation tank, flow distributor tank, and in the filters (Table 2). In the filters, samples were taken in the different layers of the bed to estimate the effect of removal through the height of the bed.
Each parameter was measured using different techniques and frequency (Table 3): Turbidity was measured twice a month (a total of 8 measurements per point), pH was measured only twice at all points because the data did not have important fluctuations. Fe and Mn were measured five times, once the system guaranteed the operation in the effective filtration phase. Dissolved oxygen (DO) samplings were also carried out to analyze their behavior during the day and in each wetland, in order to verify whether Fe and Mn were oxidizing. Flows were also measured to identify possible losses due to evaporation or leakage in the system.
Table 3 Parameters of water quality measured in certified laboratory. Source: APHA, AWWA & WEF (2012).
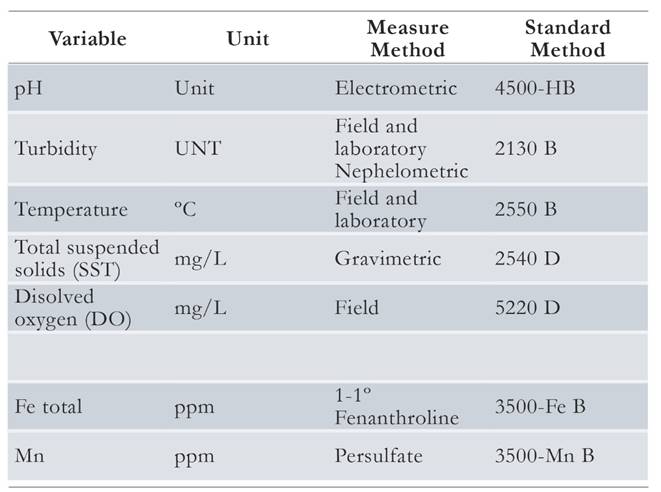
Likewise, samples of effluents from the filter backwashed of drinking water treatment plant were taken for the column sedimentation test (Huisman, 2004), to estimate the sedimentation speed of the particles and their size. The column consisted of five faucets located at the following heights: the first one at 2.5 cm from the bottom, the second at 13 cm, the third at 22.5, the fourth at 37.5 and the fifth at 57.5 cm. For the test, a sample of the wastewater of 30 gallons was taken after 10 min from the maintenance start, since at this time turbidity had the highest values. Once the water was in the sedimentation column, it was slowly mixed to begin the test, and then it was left to repose to establish the removal efficiency values and sedimentation rate. Samples were simultaneously taken with intervals of 15 minutes and turbidity was measured to determine the percentage of repulsion, and subsequently the removal efficiency in time. The sedimentation rate was determined according to Equation 1.
Where:
Vs = sedimentation rate in cm/s, Hi = height of each key, and t = time elapsed from the start of the test.
Once the sedimentation rate hadbeen calculated, the efficiency ofthe removal of sediment particles was determined by Equation 2. The procedure for calculating the percentage of removal was carried out in accordance with (Hiusman, 2004).
Where:
R = percent turbidity removal, Po = Percentage of the turbidity fraction in time over the initial turbidity, VSo = sedimentation rate corresponding to Po and Vs = critical sedimentation rate to a Dp.
The same samples taken in the sedimentation column test were used to perform total suspended solids (SST) analysis and to review some type of correlation with turbidity. Additionally, to determine the volumetric percentage distribution of a particle size, a sample of the water used in the sedimentation column test was taken, and laser particle analysis was performed using the equipment Hydro2000 SM, for particle sizes for a range between 0.02 /dm - 2 000 Also, the sludge density was estimated and a sample was collected with the resulting sludge at the end of the column sedimentation test. These were transferred to an Inhoff cone for one hour, then the supernatant water was discarded and the volume of the sludge was passed to a crucible which underwent drying. The dry sludge was transferred to even pycnometer at room temperature graduated from 0.05 L and weighed in analytical balance, then water was added to complete the volume and was reweighed. The volume of sludge was estimated with the difference between the volume of the bottle and the volume of water with sludge. The amount of dissolved sludge was determined by subtracting the weight of the pycnometer and the weight of the pycnometer with dry sludge. At the end, the weight of the dry sludge was divided by the sludge volume.
The determination of the removal percentages of Fe and Mn was calculated with the ratio of the raw water that enters the system minus the average concentrations values of effluent treated divided the average concentrations values of effluent treated multiplied by 100. For the determination of the absorption of Fe in different parts of the plants, Fe (1.10 phenanthroline) analyses was made with a Shimadzu A.A.-6300 atomic adsorption equipment and the Wizard software. After the day 172 when the pilot system was disabled, a sample of each of the three tissues (leaves, stem and root) was taken, with a mass equivalent of 2 g per tissue of each plant for a total of 9 samples per plant. To prepare the samples of the parts of each plant (tissue) the method described by (Saavedra & Rondon, 2009) was used, leaving the part of the plant to dry, then introducing it into the flask, then undergoing wet digestion in nitric acid and peroxide hydrogen. To avoid the alteration of roots sample to which Fe adheres, the roots were washed and the external tissue was removed.
The pilot-scale study was of an experimental type with a single factor in random blocks and with three levels (2 vertical wetlands + 1 control or reference wetland). The response variables were changes in concentration of Fe, Mn, Turbidity, DO and pH. For data processing, the Kolmogorov-Smirnov test was performed to determine whether the data from the different samples has a normal distribution. When carrying out this test, the P-value > 0.05 was obtained, which determined that the distribution is normal in all cases, so it was possible to apply parametric tests. Therefore, an analysis of variance (ANOVA) was carried out with a statistical package. The mathematical model applied in the experimentation of clustered blocks was as follows:
Where:
yij = Response Variable: Efficiency in iron, manganese, turbidity, dissolved oxygen
μ = General average without considering any treatment
T i = Effect of the I-th level of the wetland type factor
β j = Effect of the J-th block; each sampling section
ε ij = Experimental eError
Resulsts and discussion
The influent in the wastewater treatment plant had a pH that varied between 6.8 and 7.5 at mean water temperature of 18 ◦ C ± 2 ◦ C, also the maximum concentration of Fe and Mn presented was 4 mg/L and 2.4 mg/L, respectively, and the sludge presented a density of 1.261 mg/L. The dissolved oxygen (DO) presented concentrations of 1.26 to 2.04 mg/L, which correspond to hypoxia condition since DO is less than 5 mg/L (Bain & Stevenson, 1999) (Fig. 2A). The latter did not facilitate the complete oxidation of the Fe (II). Regarding turbidity of wastewater, it tends to rise in the beginning, but then it lowers until it reaches to stabilize its value. The peak value is between 290 and 300 UNT while the minimum values range from 1.0 to 2.5 UNT (Fig. 2B). Also, at higher superficial rates (Vsc high) less is the efficiency of removal of sediment particles (Fig. 2C), on that basis a sedimentation rate of 4.167 x10-5 m/seg corresponds to an efficiency of 85 %. The average particle sizes were 18.6 p m, being close to the calculated value for the sedimentation speed selected (Fig. 2E). The diameter of sediment particles of wastewater oscillates between 1 and 100 p m but the highest % of particles corresponds to diameters between 6-20 p m (40 %) and between 20-70 p m (40 %). Additionally, the results from the sedimentation column showed a direct correlation between turbidity and SST with R2 0.98. Given the foregoing, it was possible to evaluate the total suspended solids through turbidity as a controlled system (Low etal., 2011). For the maximum turbidity value of 240 UTN, the SST concentration was 216 mg/L and for the minimum value of 4.8 UTN it corresponded to a SST concentration of 1 mg/L (Fig. 2D).
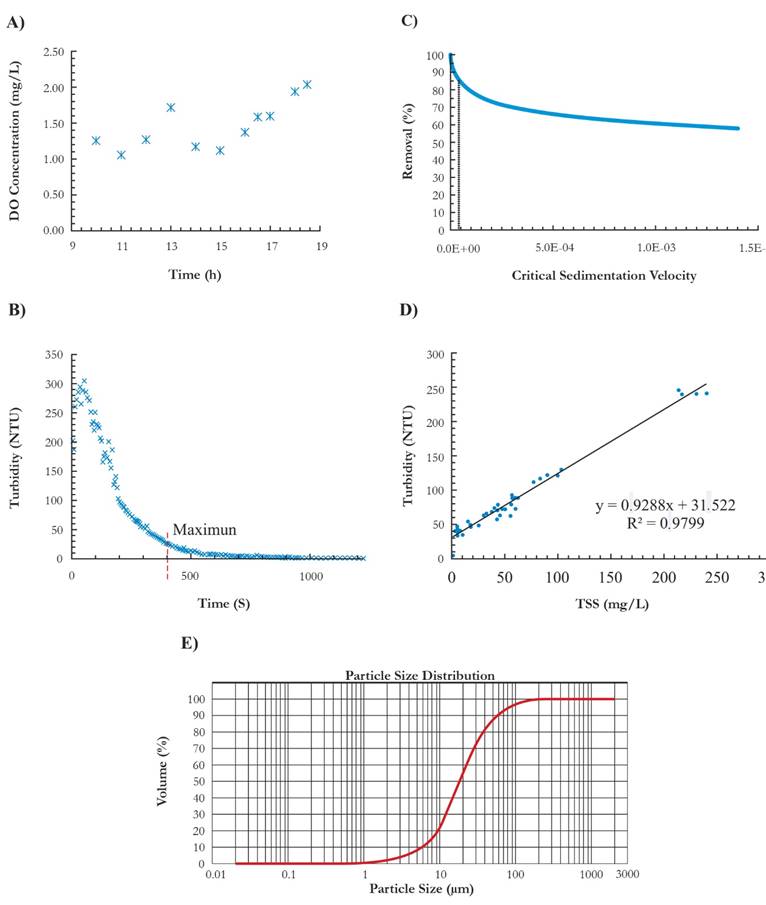
Figure 2 Characteristics of wastewater influent. a). DO concentrations at the discharge point of the treatment plant. b). Turbidity behavior. c). Relationship between the removal % and the sedimentation rate. d). Turbidity correlation with SST. e). Laser granulometry.
The pH through the filtering beds was close to neutrality with a median value of 7.75 (Fig. 3A), which is consistent with the findings of (Lopez et al., 2002), who stated that under aerobic conditions pH > 6 the oxidation of the Fe is of predominance abiotic, while in the Mn this predominance is given at pH > 8. In each wetland, the results of turbidity show maximum values of influent turbidity of 128 UNT and minimums of 37.4 UNT, indicating that considerable fluctuations were given in the values by offsets in the washing frequencies of the filters. However, each wetland had a high efficiency by presenting average removal values of 98 % in the three types of wetlands (Fig. 3C). This is consistent with the results obtained by Solis et al. (2016), who reported maximum removal values of 97 % in subsurface wetlands. The average of DO in raw water that entered the system was 3.63 mg/L and the value entered in each of the wetlands was 3.36 mg/L, 3.14 mg/L and 2.86 mg/L in the wetlands of C. esculenta, P. australis and white, respectively. The output concentrations were 2.58 mg/L, 2.1 mg/L and 2.09 mg/L in C. esculenta, P. australis and reference , respectively (Fig. 3B). It is observed that at the beginning of the day the concentration of DO is higher, but its concentration decreases in the afternoon in the wetlands with plants; a peak was also observed around 1:00 pm, time in which there was direct and higher radiation on wetlands. The highest amount of oxygen in the hours of direct radiation can be explained by the effect of oxygen supply to water by the plants (Luna & Castañeda, 2014); the plants macrophits, the emergent among them,(P. australis) and the terrestrial Hygrophytes (C. esculenta) have developed adaptation mechanisms to saturation conditions like the Aerenchyma , which allows the convection of gases until the roots in the plant, then these release the oxygen to the medium, generating a surrounding aerobic microenvironment (Xiu-Qin et al., 2014). When observing the behavior of the two wetlands it is perceived that the most stable corresponds to the planting with P. australis. The lowest DO values are also present in the hours of more radiation in the target, apparently by the heating of the upper bed which did not have upper water sheet since it was 5 cm below the surface. This warming can generate the release of dissolved oxygen by temperature increase (Munoz et al., 2015), something similar could happen in the wetland with C. esculenta because it had more exposed bed area than that of P. australis. Finally, the reference wetland showed the greatest DO decrease between bed 2 and 3, possibly due to the effect of the temperature increase; in addition to the fact that beds with macrophytes plants often diffuse oxygen in the surrounding substrate, generating favorable conditions for the oxidation of Fe and Mn (Collins et al., 2004).
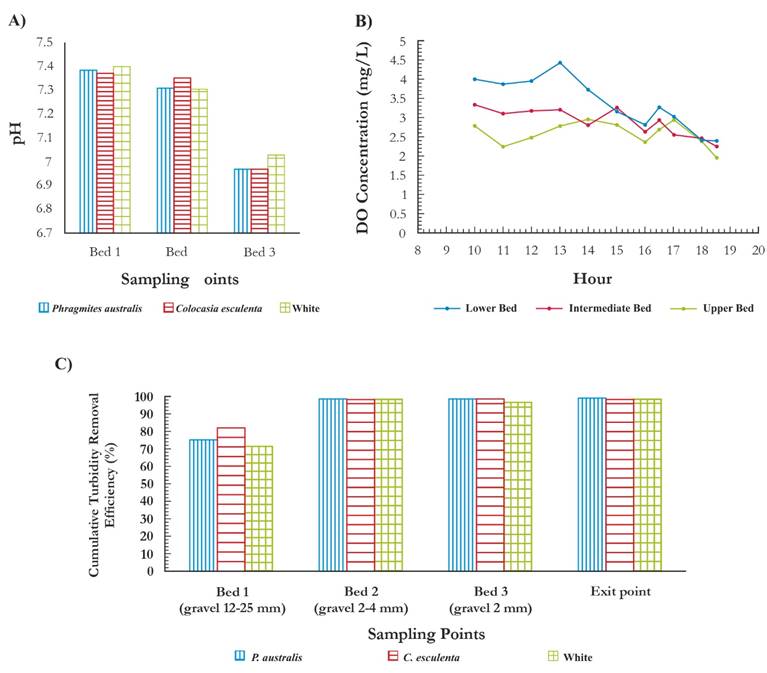
Figure 3 Characteristics of wastewater in filtering b eds. a) pH behavior in wetlands. b). DO Concentration in wetlands. c). Turbidity removal in different wetland beds.
The concentration of total Fe in the influent oscillated between 3.67 mg/L and 3.87 mg/L with a standard deviation of 0.16. Also the concentration of dissolved Fe was 0.2 mg/L. The removal efficiencies average of total Fe was 90 % and 93 %, respectively, and for dissolved Fe was 86 % (reference wetland) and 95 % (with plants), respectively. In wetlands with plants, the highest total Fe efficiencies corresponded to concentrations of 3.77 mg/L and for dissolved Fe of 0.26 mg/L. (Fig. 4A). The concentration of Mn in the influent oscillated between 2.65 and 1.37 mg/L with a standard deviation of 0.41. The removal efficiencies of Mn in all wetlands were obtained between 95 % and 96 %. In wetlands with plants, the highest total Mn efficiencies corresponded to concentrations of2.6 mg/L (Fig. 4B). Therefore, the effluent accomplished the Colombian regulations (decree 1076 of 2015, resolution 0631 of 2015 and decree 050 of 2018), since it has Fe and Mn concentrations lower than 1 mg/L and 0.2 mg/L, respectively. The results of the removal effectiveness of Fe and Mn with wetlands are consistent with those reported by Lopez et al. (2002).
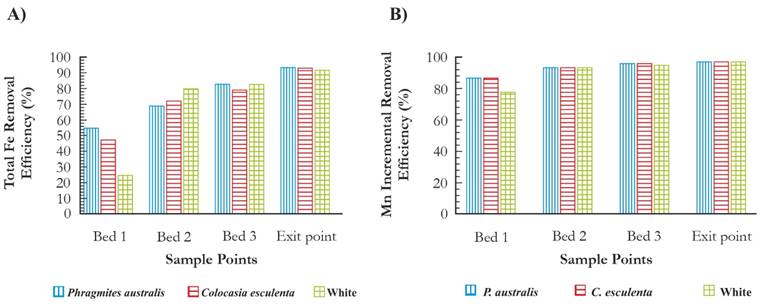
Figure 4 a). Incremental efficiency of total Feremoval in wetlands. b). Efficiency of Mn incremental removal in wetlands.
The statistical analysis with the Kolmogorov-Smirnov test showed that the data of Fe, Mn, DO and turbidity had a p-value > 0.05, indicating its normal distribution. The ANOVA analysis determined that the degrees of freedom do not correspond to the number of samples but to the grouped blocks due to the interactions between the groups. The removal of Mn, total Fe, Fe (II) and turbidity did not show significant differences between the plants and the evaluated wetlands (p-value > 0.05) (Table 4), so the difference between radicular developments did not have a relevant effect on the removal of Fe and Mn. The DO presented a significant difference (p-value < 0.05) with respect to the wetland reference, possibly due to the incorporation of DO by the plants with the photosynthesis and radial oxygen loss.
The removal of Fe and Mn in wetlands might vary due to the presence of vegetal species, the composition and characteristics of the microbial activity, as well as physical and hydrodynamic characteristics such as depth (Collins etal., 2004), location and temperature (Wang etal., 2017). However, Fe and Mn in wetlands present a change in the sediment interface by oxidation of Fe (II) and Mn (II) to Fe (III) and Mn (IV), which can be retained by sedimentation (Goulet & Pick, 2000). The highest removal efficiency of Mn in all wetlands is presented in the first bed, possibly by adsorption processes on the gravel. The removal of Mn is possible in the pH conditions, in which the pilot system operated (between 6.9 and 7.4) and may be associated with adsorption phenomena due to the presence of iron oxides on the gravel grains. For the long operation period of the pilot system it is possible that oxidizing bacteria of the Fe and Mn, contribute to the removal by their ability to deposit hydroxides of Fe or Mn oxides both intracellular and in their structures outside the cell. The optimal conditions for the growth of these bacteria are characterized by the pH and potential of oxide reduction. In the case of removal of the Mn, the optimum pH range is between 7.5 - 8.5 (Vidoni et al., 2010), so it is probable that the oxidation of Mn has also been biotic.
The efficiency Fe removal in the lower bed varied between 40 % and 50 %, possibly by retention in the filter bed of coarse particles of iron hydroxides that were not removed in the settler and were deposited in the first bed by effect of Ionic exchange with gravel or by chemical precipitation, due to oxidation. It can also be associated with the effect of a better condition for the oxidation by the development of the roots, which can contribute to a greater oxidation of the Fe in the gravel beds. Phenomena of biotic absorption or oxidation of Fe in the roots may also have contributed to such removals in accordance with (Lopez et al., 2002). This phenomenon can occur in the P. australis, which reached a root depth of 65 cm covering almost all the beds. In the C. esculenta it only reached a depth of 35 cm and this effect can be reduced, basically by the radial oxygen loss (oxygen that the plant can release through the roots in saturated water conditions) (Vymazala & Svehlab, 2013).
The radial oxygen loss can also generate significant changes in pH and dissolved oxygen in the rhizosphere, which may explain the small decreases in pH and DO. According to Xiu-Qin et al. (2014) the loss of radial oxygen promotes the oxidation of dissolved Fe by generating a plate-shaped precipitate over the roots of the plants. It is to be noted that the absorption analysis of the iron in the plants of the wetlands was made by taking samples of different parts of the plants to determine the fixation capacity of Fe. The epiphytes can absorb metals through their roots, rhizomes and leaves, because they have large areas of contact in the system of fibrous roots. Its accumulation capacity can occur even at concentrations greater than those found in the surrounding water (Bonanno & Lo Giudice, 2010).
However, the analyzed plants had a different behavior in the absorption of Fe, while the C. esculenta presented the largest fixations in the root with an average of 103.5 μg/g root, the P. australis presented the highest concentrations in the stem and leaves with averages of 41.4 μg/g stalk and 45.7 μg/g of leaves, respectively) (Fig. 5). In the C. esculenta, higher values were presented at the root compared to the rest of tissues; possibly this is associated to the tendency of this plant to accumulate the largest quantity of substances required for its development in the cormorants and root area; but, it is also probable that the endodermis of the root may act as a partial barrier to the movement of the metal between the root and the aerial part, as stated by Kabata-Pendias (2010) when analyzing the absorption of lead. Also, the distribution of metals in different tissues depends on the dynamics of each plant and the predominance of the different phytoremediation methods (Bello et al., 2018; Tuzen et al., 2018; Tka et al., 2018; Jabli et al., 2017). Consequently, it can be considered that in the C. esculenta predominates the phytostabilization of Fe fundamentally in the roots, whereas, in the P. australis predominates the phytoaccumulation, presenting a translocation of the metal with more accumulation in aerial tissues. Nevertheless, no emergent wetland plant has been identified as a hyper accumulator, suggesting that their main known function is the provision of organic matter through regressive death, and the provision of organic compounds through the root by exudation (Lizama, 2014). Less than 2 % of the metals are stored in the biomass of the microphytes, so they do not generate a significant retention of metals and only a small amount of the metals absorbed are usually transported to the roots (Marchand etal., 2010). Additionally, the phytostabilization and the phytoaccumulation of Fe suggest that once these plants are harvested, their disposal can pose a risk to the environment. The most suitable disposal treatments for these residues are incineration or using them to replace metal deficient sources, which is facilitated due to the low biomass production (Peña, 2013; Batista & Sánchez, 2009). However, further research is neededin this area, taking into account the precautionary principle that makes it necessary to limit its contact with animals and harvestable plants.
Although there were no significant differences in the removal of Fe and Mn by the analyzed plants, the load loss curves for each wetland showed that the maintenance frequency of the wetland with C. esculenta is higher, followed by the wetland with P. australis, and finally the reference (Fig. 6). The latter might be explained by the type of root development of each plant, since P. australis has thin roots that grow mainly in vertical direction, while C. esculenta has a thicker root (higher roots density) and a radial growth. The less load loss of the reference wetland is explained by the non-presence of plants, which generates less resistance to the flow. Therefore, it is advisable not to use plants to reduce maintenance, however, further research is recommended for the use of plants in the wetlands because they reduce, remove, transform, degrade, mineralize, volatilize or stabilize substances that due to their concentration, permanence or location may represent a contamination hazard (Delgadillo et al., 2011). Likewise, research on local plants should be prioritized, since species such as P. australis are considered an ecological hazard due to their rapid spread and expansion. If it arrives in wild aquatic ecosystems it could alter their hydraulic regimes, displace native species and increase the risk of fire (Swearingen & Saltonstall, 2010). In this field there are still major research challenges and an important limitation, because without the use of plants or some technologies to remove or reduce the pollutant load of the wetland, each drinking water treatment plant will require a treatment system for wastewaters, which in turn will require a treatment system for wastewaters and so on. For now, the design of the pilot plant could present a temporary solution by using filters in parallel, which could be alternately washed to incorporate the effluent to the other filters.
Conclusions
The effectiveness of vertical subsurface wetlands for Fe and Mn removal from wastewater in drinking water treatment plants was confirmed, especially for waters with concentrations of Fe between 3.6 and 3.9 mg/L, and Mn between 1.8 and 2.6 mg/L, with pH values between 6.5 and 7.4 and dissolved oxygen less than 4.5 mg/L. The efficiency removal was about 90 % of Fe, 97 % of dissolved Fe and 98 % of Mn. However, the biggest removal is attributed to the filtration processes in the gravel and not by absorption in the plants (C. esculenta and P. australis) tissues.
Therefore, sub-surface wetlands can be an interesting alternative for the treatment of wastewater from the maintenance of drinking water treatment plants. However, research on these wetlands should prioritize the study of native species for the phytoremediation of metals such as Fe and Mn, as there is an important limitation in wastewater treatment with wetlands, since, without the use of plants or some removal technologies the pollutant load will continue in this system . A temporary solution, could be the use of filters in parallel, incorporating the effluent of a washed filter into the other filters.
Finally, detailed analysis is recommended for the selection of a sub-surface wetlands technology for wastewater treatment from drinking water treatment plants depending on the context, taking into account the characteristics of the wastewater, the available macrophyte plants, the environmental conditions and especially the local costs that may vary. Likewise, it is necessary to consider that elements such as Fe and Mn can be mobilized and accumulated in the tissues of the plants or adhered to their surface, so the safe way to dispose these residues should be considered from the design.