Introduction
Greenhouse gas (GHG) emissions, primarily composed of carbon dioxide (CO2), methane (CH4), and nitrous oxide (N2O), pose a significant global challenge. Notably, CO2 accounts for nearly 75% of global GHG emissions, with fossil fuel combustion alone contributing 65% 1. This situation is alarming, as the rapid accumulation of atmospheric CO2 can lead to ocean acidification, extreme weather events, accelerated continental ice melt, and other potentially irreversible consequences 2,3. In response, the UN Emissions Gap Reports of 2019 and 2020, along with the IPCC-SR15 Report of 2018, urge an urgent 55% (32 GtCO2e) reduction in CO2 emissions by 2030 to limit global warming to 1.5°C, in line with the 2015 Paris Agreement 4-6. Achieving these global goals requires implementing multifaceted strategies to reduce atmospheric carbon levels, including a rapid shift from fossil fuels to renewable energy sources, carbon capture and storage (CCS), improving fossil fuel energy production efficiency, and better management of terrestrial CO2 sources and sinks (the natural carbon cycle) 7.
Among non-conventional renewable energies (NCRE), biomass is considered an abundant and approximately carbon-neutral energy resource, as CO2 is removed from the atmosphere and solar energy is stored through biomass formation via photosynthesis. However, biomass-to-energy conversion processes can still be major point sources of CO2 previously sequestered during biomass growth. To address this, carbon capture and storage (CCS) technologies, originally designed for the fossil fuel industry 8-10, can be integrated into these biomass-to-energy conversion processes, such as in biomass refineries or biomass gasification plants 11. The captured CO2 can then be stored in suitable geological reservoirs or utilized for other purposes 12. This is known as BECCS (bioenergy with carbon capture and storage) technologies. The development of BECCS will have a significant impact on bioenergy markets, enabling the targeting of fossil fuel-intensive sectors such as transportation, energy, and industry 13. Additionally, the introduction of biogenic CO2 storage and utilization can significantly contribute to circular economy strategies 14,15.
Prior to large-scale national deployment of BECCS technologies, large-scale agro-industrial enterprises with high BECCS efficiencies are emerging as the first movers to achieve deep reductions in atmospheric CO216. Such is the case with energy-driven biorefineries, where the ultimate goal is the production of energy in the form of biofuels, electricity, and/or heat 17, playing a vital role in decarbonizing the energy matrix. A particular case is sugarcane plantations, which have an impressive rate of atmospheric CO2 drawdown and, when processed in bioethanol biorefineries (producing bioethanol and thermal/electric energy) with CCS technologies, can achieve highly negative emissions.
Agriculture plays a significant role in Colombia's economy, contributing 6.3% to the country's GDP. Over 200 different crops are cultivated annually, utilizing approximately 4.4 million hectares of land to produce over 49 million tons of agricultural products. Thirteen crops account for over 90% of agricultural production, with sugarcane being the most significant, representing 49% of total output 18. The sugarcane industry is a prime example of the convergence of agro-industries towards biorefinery models. Given the importance of agriculture and the potential of BECCS technologies in sugarcane biorefineries, this bibliometric analysis aims to examine the current state of BECCS implementation in Colombia and its alignment with national energy transition and circular economy policies.
Metodology
The bibliometric review was based on scientific articles published in the SCOPUS database with the following keywords: "BECCS (Bioenergy Carbon Capture and Storage)", "Bio-energy with carbon capture and storage (BECCS)", "bioenergy CCS (BioCCS)", "Bio-CCS", "Biopower CCS", "Bio energy with CCS". To focus the search, the following filters were used: document type: articles and time limit: 2011-2021. In Figure 1, the research dynamics (evolution over time) of the 451 identified scientific publications are shown, where a pronounced and sustained growth is observed from the year 2016.
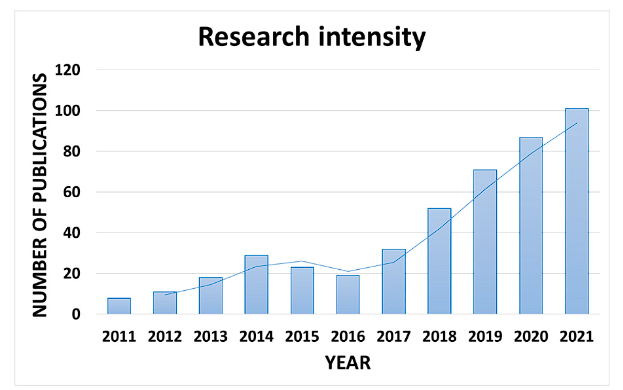
Figure 1 Research intensity on the BECCS topic over the last 10 years (SCOPUS database, search criteria: TITLE-ABS-KEY (({BECCS} OR bioenergy OR {Bio-energy} OR {Bio energy} OR {Bioenergy Carbon Capture} OR {BioCCS} OR {Bio-CCS} OR {Biopower CCS} OR {bioenergy CCS}) AND ((carbon AND capture AND storage) OR (CO2 AND capture AND storage))) AND PUBYEAR > 2010 AND PUBYEAR < 2022 AND (LIMIT-TO (DOCTYPE, "ar")).
From the perspective of fields of study, it is observed that "Environmental Sciences" predominate with 299 publications (29% of the total), "Energy" with 251 publications (24% of the total), and "Engineering" with 117 publications (11% of the total).
As a result of this bibliometric review, the evaluation of BECCS potential carried out by different countries (Australia, Brazil, Finland, Sweden, Denmark, Korea, Austria, North America, China, Japan, among others) stands out, and the technologies used for CO2 removal are based on physical and chemical techniques. Chemical CO2 adsorption technologies based on amines are presented as the most developed technology for combustion CO2 capture (1st generation). Meanwhile, among the second-generation options for CO2 capture in biomass co-generation (bioelectricity and/or heat), chemical looping processes (CLP) have emerged in recent years as a competitive option, as they achieve higher energy efficiency by inherently avoiding gas separation steps (CO2 is captured intrinsically), and they can be used in oxy-combustion and pre-combustion processes.
Based on the potential of the CLP technique in capturing CO2 derived from the biomass co-generation process, this bibliometric review identified some critical elements of the technology, with the main one being "solid oxygen carriers," detailed in the following section.
Results and Discussion
International Context
There is global consensus that CO2 plays a key role in global warming. This is because CO2 absorbs outgoing infrared radiation from the Earth's surface and re-emits part of it back to Earth, accounting for 65% (1.7 W/m2) of the total global downward radiative forcing of 2.6 W/m2 for all greenhouse gases combined 19. Therefore, the rapid accumulation of atmospheric CO2 can lead to ocean acidification, extreme weather phenomena, rapid melting of continental ice, among other consequences 2-3, which can be irreversible. It is concerning that the average annual growth rate in atmospheric CO2 concentration has steadily increased from 0.9 ppmv/year in the 1960s to 1.5 ppmv/year in the 1990s and to almost 2.5 ppmv/year in the 2010s 20. Given this situation, in December 2015 at the 21st Conference of the Parties to the United Nations Framework Convention on Climate Change (UNFCCC) in Paris, 147 countries established a global aspiration to limit the temperature increase to 1.5°C above pre-industrial levels 21, thereby avoiding irreversible changes in our planet's biosphere. To achieve this limit, achieving net zero carbon emissions by 2050 will be required, as well as removing 100 to 1000 GtCO2 from the atmosphere during the 21st century 22. However, the United Nations Emissions Gap Report 2019 4 warned that with nearly 55.3 GtCO2e of emissions in 2018 (2/3 of the total global greenhouse gas emissions), the world is on track for a 3.2°C temperature increase.
The energy generation sector primarily driven by fossil fuels (80%) accounted for 36% of CO2 emissions in the world's advanced economies in 2019, down from 42% in 2012 23-24. Although the share of coal in primary energy in 2019 fell by 15%, it remains an important source for the global energy mix, maintaining a steady flow of CO2 emissions 23. While during the COVID-19 lockdowns in 2020, it was predicted that world CO2 emissions would decrease by about 7% compared to 2019 emissions 25, the impact was quite limited 26. In fact, in 2020, global energy demand fell by 4%, resulting in a reduction of ~2 GtCO2 (5.8%) in global CO2 emissions of 31.5 GtCO2, but this quickly reversed to recover with a 4.8% increase in CO2 emissions by late 2021 27.
Given the critical situation described, reports on the United Nations emissions gap in 2019 4 and 2020 5, as well as the Intergovernmental Panel on Climate Change (IPCC) Special Report on Global Warming of 1.5°C (IPCC-SR15) in 2018 6 urgently call for a 55% reduction (32 GtCO2e) in CO2 emissions by 2030 to limit global warming to 1.5°C, in accordance with the 2015 Paris Agreement. Furthermore, the Group of Seven (G7) countries in 2021 committed to collectively reduce their emissions by 50% by 2030 and achieve net zero emissions before 2050 28. To achieve these goals on a global scale, multifaceted strategies must be implemented in parallel to reduce atmospheric carbon levels, such as rapidly transitioning the energy supply from fossil fuels to renewables, capturing and storing CO2 (CCS), improving efficiency in fossil fuel energy production, as well as better managing sources and sinks of CO2 on land (natural carbon cycle) 7.
From the perspective of renewable energies (biomass, solar, hydro, wind, geothermal, and marine), their contribution to the aforementioned climate goals would be achieved by increasing their share in global energy supply to around 75% by 2050 29. Despite the economic slowdown induced by COVID-19, the world added over 260 gigawatts (GW) of renewable energy in 2020 to a total of 2799 GW, surpassing the expansion in 2019 by almost 50% 30, and representing a 29% share of global energy supply 31, indicating progress is being made.
Within unconventional renewable energies (URE), biomass is considered an abundant and approximately carbon-neutral energy resource. CO2 is removed from the atmosphere and solar energy is stored through biomass formation via photosynthesis. This means that the use of biomass as fuel (solid, liquid, or gas) or as a vector in bioenergy generation 32 does not significantly increase the total atmospheric CO2 inventory 33, besides directly or indirectly impacting various Sustainable Development Goals (SDGs), including SDG 7: Affordable and Clean Energy, SDG 13: Climate Action, SDG 15: Life on Land, SDG 8 - Decent Work and Economic Growth, and SDG 5 - Gender Equality 34. Although total bioenergy production in 2020 was 126 GW (4.5% of total renewable energy produced) 30, it is expected to significantly contribute to the global primary energy matrix by 2050 (increasing its share from 2 to 6 times) 35, and play a fundamental role in sectors that are difficult to electrify, such as maritime transport, aviation, and certain industrial processes 29. However, bioenergy plays a key role in the European Union's (EU) 2030 goals of at least a 40% reduction in greenhouse gas emissions compared to 1990 levels, over 32% of renewable energy share in final energy consumption, and over 32.5% improvement in energy efficiency 36. In the case of China, bioenergy's share in the national energy matrix is projected to triple from 3% in 2020 to 9% in 2060, and it will represent almost 7% of CO2 emission reductions by 2060 37. It is also expected to replace 30% or more of US oil consumption by 2030 38.
Given that biomass conversion processes into energy can still constitute significant point sources of CO2 previously sequestered during biomass growth, carbon capture and storage (CCS) technologies, already implemented or in development for the fossil fuel industry 8-10, can be integrated into these, for example, in biomass refineries or biomass gasification plants 11, to reduce biogenic CO2 emissions to the atmosphere 39. In this way, captured CO2 can be stored in appropriate geological reservoirs or used for other purposes 12. This is known as bioenergy with carbon capture and storage (BECCS) technologies.
The advantages and CO2 removal potential encompassed by BECCS have made it the dominant "negative emission technology" (NET) in Integrated Assessment Models (IAMs) of the Intergovernmental Panel on Climate Change (IPCC) 40-41. Thus, BECCS is an essential part of most modeling pathways that limit global warming to 1.5°C, projecting the removal of between 151 and 1191 Gt of CO2 worldwide during the 21st century from 2020 42. These targets correspond to removing an average of 22.5 GtCO2/year 43, equivalent to over 1/3 of the global CO2 emissions reported for 2019 44. In other comprehensive studies based on different options for carbon removal from the atmosphere using NET, including BECCS, afforestation and reforestation, direct air capture and storage, enhanced weathering, ocean fertilization, biochar, and soil carbon sequestration, it was concluded that BECCS technologies represent a good compromise between CO2 removal potential (0.5-5 GtCO2/year) and CO2 removal costs of 100-200 USD/tCO245. It is worth noting that the development of BECCS will influence bioenergy markets, as it will enable addressing other segments apart from the transportation sector, such as the energy 13 and industrial sectors, where CO2 capture and storage technologies have been intensively developed over the last 20 years 46. Furthermore, the introduction of biogenic CO2 storage and utilization can significantly contribute to circular economy strategies 14-15. Given the potential represented by BECCS, it is not surprising that research intensity over the last 10 years has increased substantially (see Figure 1).
Based on the above, examples of BECCS potential assessment carried out by different countries can be found in the literature. Australia focuses on organic waste from municipal, agricultural, and forestry sectors 47. Brazil identified the possibility of cost-effective negative emissions in the joint production of ethanol and electricity from sugarcane, bagasse, and other residues 48. In the Nordic countries (Finland, Sweden, and Denmark), per capita biomass potential is one of the largest in the world, facilitating the development of biomass-based BECCS 49. The use of domestic forest biomass as a source of bioenergy is also considered an option in Korea 50 and in Austria 51. In North America, BECCS based on forestry, agricultural residues, and dedicated energy crops can enable a negative carbon energy system in the western region by 2050, with emissions reduced by up to 145% compared to 1990 levels 52. In China, with 73% of national crop residues used between 2020 and 2030, and utilizing BECCS technology, an accumulated reduction of GHGs of up to 8620 Mt CO2-eq by 2050 could be achieved, contributing between 13% and 31% of the global GHG emission reduction target for BECCS, and almost 4555 Mt more than projected solely for BECCS in China 53. Japan, on the other hand, has technically and economically evaluated the potential of using woody biomass with BECCS technology, bearing in mind that around 70% of the country's land mass is covered by forests 54.
Before the deployment of BECCS technologies at a country scale, large-scale agro-enterprises with high BECCS efficiencies emerge as the first agents to achieve deep reductions in atmospheric CO216, such as energy-driven biorefineries where the ultimate purpose is energy production in the form of biofuel, electricity, and/or heat 17 playing a vital role in decarbonizing the energy matrix. A particular case is sugarcane plantations, which have an impressive performance in atmospheric CO2 drainage rate and, when processed in bioethanol biorefineries (bioethanol production and thermal/electric energy) with CCS technologies, can achieve highly negative emissions. This is possible because the CO2 from the fermentation stage in bioethanol production is practically pure 55-56. It has been calculated for Brazilian sugarcane biorefineries the potential immediate removal of 27.7 MtCO2/year through BECCS in the fermentation stage 48, meanwhile, the bagasse-fed cogeneration system can represent approximately 5 times the amount of CO2 emitted in the fermentation process 57-58. In this regard, given the impact of cogeneration on CO2 emissions, a special technological focus is required to achieve efficient CO2 capture. Chemical adsorption technologies for CO2 based on amines are presented as the most developed technology for combustion CO2 capture (TRL 8-9), still presenting significant drawbacks for implementation in biomass combustion, including significant energy penalty, limited operational flexibility, critical increase in water usage, waste toxicity, and solvent degradation 59. Therefore, this CO2 capture strategy presents a low research intensity in the last 10 years, as shown in Figure 2.
Among the second-generation options for CO2 capture in biomass-fed cogeneration of energy (bioelectricity and/or heat), Chemical Looping Processes (CLP) have emerged in recent years as a competitive option. They achieve higher energy efficiency by inherently avoiding gas separation steps (CO2 is captured intrinsically) and can be used in oxy-combustion and pre-combustion processes 7-60. In fact, CLP provides the best cost reduction benefit among various current and projected Carbon Capture and Storage (CCS) technologies in a carbon-constrained scenario, according to the U.S. Department of Energy's roadmap 61. In Europe, a techno-economic study of biomass-to-energy with Integrated CO2 Capture (TESBIC) 62, comparing 28 different potential combinations of CCS technologies, concluded that CLP was a highly competitive technology, with the lowest potential CO2 reduction cost among the technologies considered. Therefore, there is a growing interest in incorporating this technology into biomass residue-fed cogeneration processes 63, such as in cogeneration plants fueled by sugarcane residues 64. Figure 3 shows the research intensity in the use of Chemical Looping Processes (CLP) in energy generation from biomass over the last 10 years. The high dynamism observed is in line with the increasing deployment of governmental initiatives worldwide in the field of energy transition, such as the EU's "European Green Deal" 65.
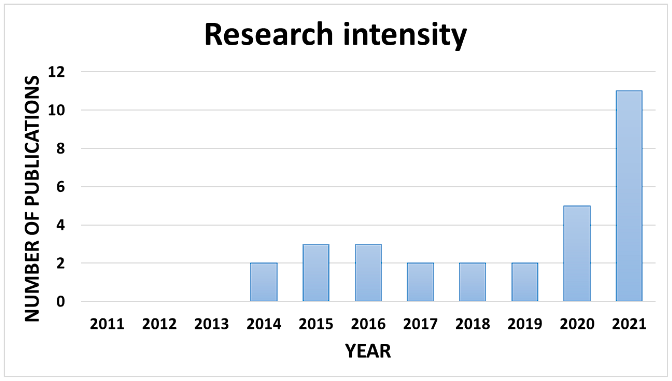
Figure 2 Research Intensity Over the Last 10 Years of CO 2 Capture from Biomass Energy Generation Processes Using Amine Adsorption (SCOPUS Database, Search Criteria: TITLE-ABS-KEY (biomass AND energy AND amine AND absorption)).
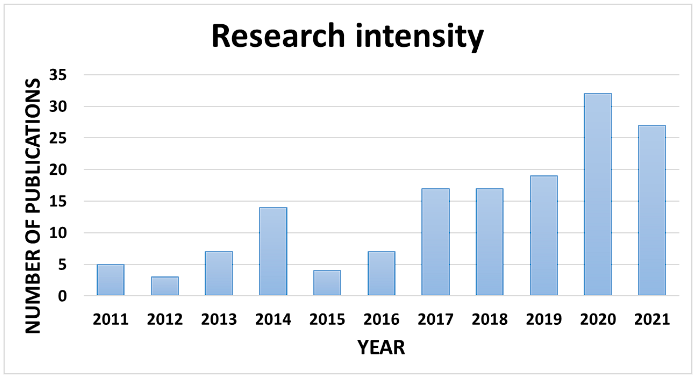
Figure 3 Research Intensity Over the Last 10 Years Associated with the Use of Chemical Looping Processes (CLP) in Biomass Energy Generation (SCOPUS Database, Search Criteria: TITLE-ABS-KEY (biomass AND energy AND {chemical looping} AND carbon)).
Two configurations of CLP are used in BECCS, namely chemical looping combustion (CLC) and chemical looping gasification (CLG) 63. In both, cyclic processes are carried out using solid materials, mainly metal oxides (MexOy) denoted as oxygen carriers (OC), which react and regenerate (reduction-oxidation) to produce a combination of heat, electricity, fuels, and/or chemicals. In this sense, the stability and performance of OC after many cycles are critical, so there is an urgent need to increase their lifetime (62). For this purpose, two routes have been explored: i) the production of OC from synthetic reagents, and ii) the conditioning of low-cost OC. Within the latter, materials based on natural minerals and byproducts of industrial processes are included 66-68. It is worth noting that the incorporation of industrial byproducts as OC contributes to circular economy strategies, and therefore to global actions for mitigating climate change 69. Regarding this, one of the most studied OCs within this approach is iron-based (Fe) due to its lower cost compared to those based on Co, Ni, Cu, and Mn, high mechanical strength, ease of handling, and environmental friendliness 70,71. Worldwide, 404 hours of operation have been reported with low-cost iron oxide material (byproducts of the iron and steel industry) from four different pilots with promising results 71. Therefore, evaluating industrial byproducts based on iron as potential OCs presents an important opportunity to impact the cost structure of the cogeneration process and environmental objectives. Figure 4 shows the increasing trend in research intensity related to the use of low-cost oxygen carriers in CLP processes for biomass energy generation over the past 10 years. This trend may be motivated in part by incentives related to circular economy policies in different countries.
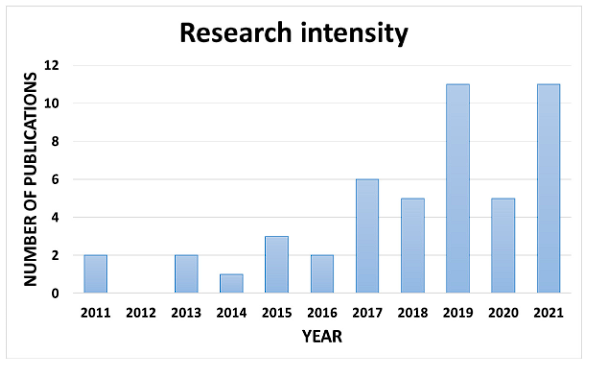
Figure 4 Research intensity in the use of low-cost oxygen carriers in chemical looping processes (CLP) for biomass energy generation over the past 10 years (SCOPUS database, search criteria: TITLE-ABS-KEY (biomass AND {chemical looping} AND oxygen AND carrier AND (low AND cost OR inexpensive OR {by-product} OR {cheap raw} OR ore OR mineral OR natural OR tailings OR {industrial residues} OR {industrial waste} OR {cheap material} OR {waste material}))).
In the BECCS process, once the CO2 is captured from the point sources (for example, the fermentation stage in bioethanol production and the cogeneration stage), it is transported to a location where it can be injected into geological storage media, such as deep saline aquifers and/or depleted hydrocarbon reservoirs. Globally, these formations are abundant and provide safe long-term storage for the permanent immobilization of CO272. However, it is estimated that 50% of CO2 geological storage projects use sandstone deposits (oil and gas reservoirs are mostly composed of sandstone) because they have technical advantages and high availability 73. The injected CO2 is retained by various trapping mechanisms (hydrodynamic, capillary, adsorption, and dissolution) 74-75. Given the importance of this stage for safely storing the captured CO2 from either bioenergy production or fossil fuel combustion, there is a growing interest in its research over the past 10 years (see Figure 5).
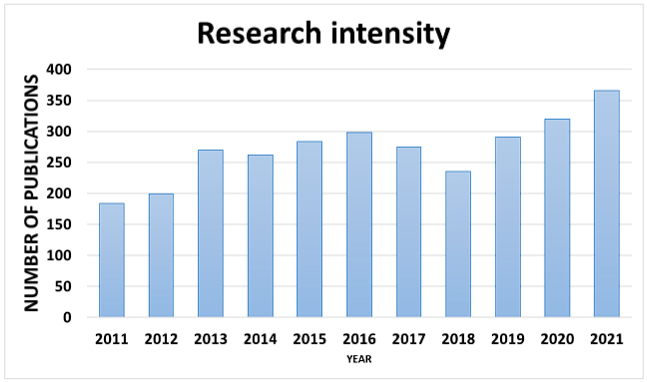
Figure 5 Research intensity associated with the study of captured CO 2 storage from bioenergy production and fossil fuel combustion in geological formations over the last 10 years (SCOPUS database search criteria: TITLE-ABS-KEY (({CO 2 } OR {carbon dioxide} OR {carbon emission}) AND (storag* OR sequestrat* OR entrapment OR entrapped) AND geological) AND PUBYEAR > 2010 AND PUBYEAR < 2022 AND (LIMIT-TO(DOCTYPE, "ar")).
In this context, the wettability of the geological rock formation governs the ability of injected CO2 to be stored 73-77, making it a critical parameter in determining the hydrodynamic and capillary trapping potential of CO2. Because the wettability of geological formations is affected by the presence of organic acids on their surface and thus their storage capacity 78, the scientific community's interest has turned towards improving this property to achieve safe and efficient CO2 storage 72. Among the enhancement strategies, the use of nanoparticles (NPs) in the form of nanofluids and surfactants stands out 79-80. However, nanofluids as an emerging research area (see Figure 6) have shown great potential to reverse the wettability of the reservoir rock to favorable conditions even in the presence of organic acids. This is crucial for reservoir modeling and the evaluation of the viability of CO2 geo-storage, including the thresholds of organic acids and nanofluids, so that better decisions can be made with less uncertainty 72. It is worth noting that various nanoparticles are used depending on the type of geological formation 81-82.
Although nanofluids have been evaluated in recent years with promising results, the types of nanoparticles (NPs) used are very limited and of synthetic origin, with SiO2, Al2O3, and ZrO2 being the most studied. There is a need to produce and evaluate other cost-effective nanomaterials 72) within the framework of the Circular Economy that, on one hand, improve CO2 storage properties in geological reservoirs and, on the other hand, contribute to global actions for mitigating climate change.
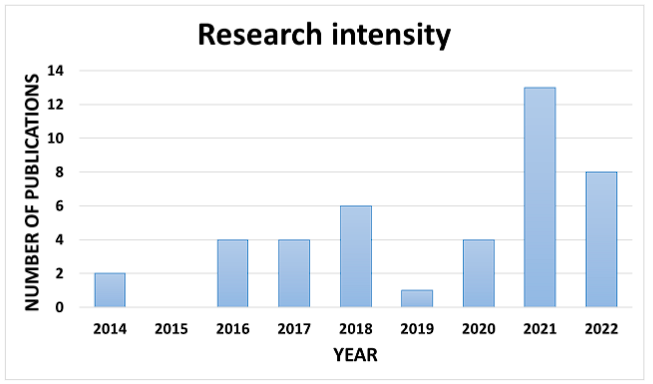
Figure 6 Research Intensity in the Use of Nanofluids for Enhancing Geological Storage Properties of Captured CO 2 (SCOPUS Database Search Criteria: TITLE-ABS-KEY (({CO 2 } OR {carbon dioxide} OR {carbon emission}) AND (storag* OR sequestrat* OR entrapment OR entrapped) AND (nanofluid OR nanoformulation OR {nanoparticle fluid} OR {nanoparticle solution})) AND PUBYEAR > 2010 AND (LIMIT-TO (DOCTYPE, "ar")).
National Context
Colombia accounted for approximately 0.4% of global greenhouse gas (GHG) emissions in 2018, which amounted to ~303 million tons of CO2 eq. This value represented a total growth of Colombia's GHG emissions by 34.7% since 1990. Livestock, agriculture, and land use change were the activities that generated the most GHG emissions (63.4%), followed by the energy sector (28.4%), waste (5.3%), and industrial processes and product use (2.9%) 83. It is worth noting that, although the global contribution of GHGs is very low, the country is highly susceptible to the effects of climate change. According to the Global Climate Risk Index, the country ranked 53rd in 2018 and 44th for the period from 1998 to 2018, showing a moderate performance against climate-related events 84.
Aware of its vulnerability and in line with global actions to mitigate climate change, since 1994 (Law 164/94), Colombia has been developing an increasingly robust and concrete regulatory framework regarding actions for monitoring, controlling, and reducing GHG emissions 85. With the adoption of the Paris Agreement, the country presented its Nationally Determined Contribution (NDC) to the United Nations Framework Convention on Climate Change (UNFCCC) in 2015, committing to reduce its GHG emissions by 20% by 2030, relative to the projected level for 2030, with the possibility of increasing its commitment to 30% with international cooperation support. It also presented some measures for adapting to the adverse effects of climate change and implementing strategies 86. With the update of the NDC presented in 2020 to the UNFCCC 87, Colombia set a more ambitious goal and committed to reducing GHG emissions by 51% by 2030 (maximum emission limit of 169.4 million tCO2e). However, the actions aimed at achieving these goals are still not sufficient. According to the Climate Change Performance Index in 2021 88, Colombia ranked 25th (medium category), given its moderate performance in actions to reduce GHGs, low performance in actions to expand renewable energies, high energy consumption, and medium performance in generating public policies associated with countering climate change.
As mentioned earlier, the energy sector is a significant contributor to GHG emissions (28.4%, 92 million tons of CO2eq in 2018). Compared to GHG emissions in 1990, this sector has experienced a strong increase of 85%. The subcategory that contributes the most is transportation (38.2%, approx. 37 million tons of CO2eq), followed by the energy industry (24.6%, 24.5 million tons of CO2eq), and fossil fuel combustion in industries (17.3%, 13.1 million tons of CO2eq). Regarding the composition of GHGs, CO2 is the gas with the highest contribution to total emissions from this sector (88.9% historical average); CH4 and N2O contributed 9.9% and 1.2% (historical average), respectively 83. It is not surprising, then, the latent need to reduce the contribution of GHGs from this sector, starting with the country's energy matrix and the transportation sector.
Historically, electricity generation in Colombia has relied on large hydroelectric plants, which are highly vulnerable to climate variations affecting water reserves. In the early 1990s (when over 80% of electricity depended on large hydroelectric plants), a severe El Niño event that reduced water reserves to below 40% triggered the country's largest energy crisis, causing significant blackouts. Subsequently, between 2015 and 2016, another El Niño event prompted an energy-saving campaign to control electricity consumption. Overall, the impacts of climate events such as "El Niño" and "La Niña," and climatic seasonality intensified by climate change, cause variability in the power capacity of hydroelectric plants between 45% and 95% of Colombian electricity generation 18. It is important to note that, in these cases, thermal plants operated by coal or petroleum derivatives have been used to assume a greater share of kilowatt-hour supply, knowing that it is a more expensive and more polluting option. It is also worth mentioning that there is still a high dependence on these two sources of energy in the country's energy matrix (68.3% hydroelectric and 30.7% thermal) 89, so the system's vulnerability remains. This situation of "electric stress" and environmental pollution has generated growing interest in the development and deployment of other unconventional renewable energies (URE) in the country (solar, wind, biomass, among others). In this regard, with the enactment of Law 1715 in 2014 90 for the development and use of non-conventional energy sources (NCES), mainly renewable energies, significant possibilities for the adoption of this type of energy were opened. This law defines public policies, tax laws, customs duties or tariffs, accounting instruments, and participation in the Colombian energy market Colombia boasts the second-largest hydroelectric potential in Latin America, after Brazil, with a potential of 5 GW for small hydroelectric plants (SHP). Additionally, the country has favorable conditions for wind and solar energy. Some areas have wind densities exceeding 400 W/m2, while the average solar radiation in the National Interconnected System (SIN) is around 4.5 kWh/m2, and the solar radiation potential in non-interconnected areas reaches 6 kW h/m2. Furthermore, the country's agricultural sector, including coffee and sugarcane plantations, has biomass and cogeneration potential 91. Regarding the latter, some studies indicate an electrical potential based on biomass of 15 GW 18. However, despite the potential and legal framework (Law 1715), the contribution of these energies to the country's energy matrix remains low. In 2020, a total of 224.3 megawatts were installed, representing only 1.3% of the matrix. According to the Ministry of Mines and Energy 89, as of 2021, Colombia had projects related to URE, including biomass, biogas, and geothermal energy, in operation, execution, or contracting phases, which would reach 2400 megawatts of installed capacity by 2022, representing close to 14% of the electric matrix in 2022. From the perspective of research intensity, Figure 7 shows a growing trend from 2014 to 2020, which can be associated with the country's public policies promoted during that period. Meanwhile, the trend in 2021 and 2022 reflects the effect of COVID-19, as mentioned earlier in the international context.
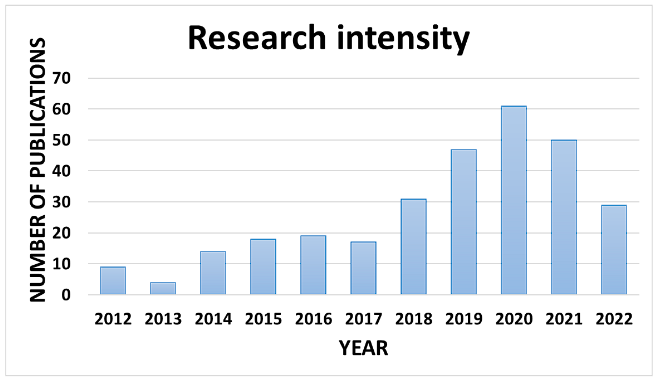
Figure 7 Research intensity on the topic of Renewable Energies in Colombia over the last 10 years (SCOPUS database, search criteria: TITLE-ABS-KEY (renewable AND energy AND colombia) AND AFFIL (Colombia)).
The agricultural sector in Colombia is a potential source of biomass, with various residual materials such as bagasse. Agriculture accounts for 6.3% of Colombia's GDP. Each year, over 200 different crops are cultivated across approximately 4.4 million hectares, producing over 49 million tons of agricultural products. Thirteen crops represent over 90% of agricultural production, with sugarcane being the most significant at 49% of production, followed by plantain (7%), potato (6%), rice (6%), cassava (4%), banana (4%), maize (3%), oil palm (3%), panela cane (2%), citrus fruits (2%), coffee (2%), pineapple (1%), and tomato (2%). These crops occupy 82% of agricultural land 18. According to reports from the Mining-Energy Planning Unit (UPME), 29 million tons/year of residual biomass are reported, with an energy potential of 12 MWh/year 92. Therefore, agriculture plays a crucial role in transitioning towards clean energy, as the use of crop residues or dedicated energy crops could support a significant amount of renewable energy production in the country 18.
Recognizing the importance of biomass, the Ministry of Mines and Energy (MME) and its affiliated agencies have adopted several policies and programs aimed at promoting the deployment of bioenergy technologies. Examples include mandatory blends for bioethanol and biodiesel (Laws 788 of 2002 and 939 of 2004 and Decree 4892 of 2011), policy guidelines for promoting biofuel production (Conpes 3510 of 2008), and programs to promote efficient and rational use of energy and alternative energies (Law 697 of 2001, Resolution 180919 of 2010, Law 1715 of 2014). Additionally, in 2018, the Energy and Gas Regulation Commission (CREG) issued Resolution 30 of 2018, which establishes rules for the free trade of electricity, based, for example, on self-generation processes. This support for bioenergy has been driven by the government's aim to generate rural employment, improve rural development, diversify the energy portfolio, reduce carbon emissions in the transportation sector, and decrease dependence on oil 93.
As mentioned in the international context, bioenergy generation processes can significantly contribute to achieving negative CO2 emissions when coupled with carbon capture and storage (CCS) technologies, a configuration known as BECCS (Bioenergy with Carbon Capture and Storage). In Colombia, studies in this field are still in their early stages, with only 2 publications related to the topic to date (see Table 1). Therefore, there is an opportunity to contribute to the knowledge and development of solutions focused within this field.
Table 1 Studies related to BECCS (Bioenergy with Carbon Capture and Storage) at the national level.
Ref. | Crop Type | CO2 Source | CCS Technology | Comments |
---|---|---|---|---|
94 | Sugarcane | Fermentation | Absorption | - Theoretical values. - Used in Enhance Oil Recovery process in conjunction with other CO2 sources (cement, steel industries, etc.) |
95 | Sugarcane, Oil Palm, Agricultural and Forestry Residues | Fermentation, Cogeneration | Not specified | - Study of scenarios exposing the potential CO2 removal with BECCS from all residues together |
On the other hand, regarding the development of energy-driven biorefineries at the national level, there has been increasing activity in experimental research and the use of computational tools in the last 5 years to assess the technical, economic, and environmental potential of using crops and agricultural residues under this production scheme. This trend aligns with the global research intensity, as illustrated in Figure 8.
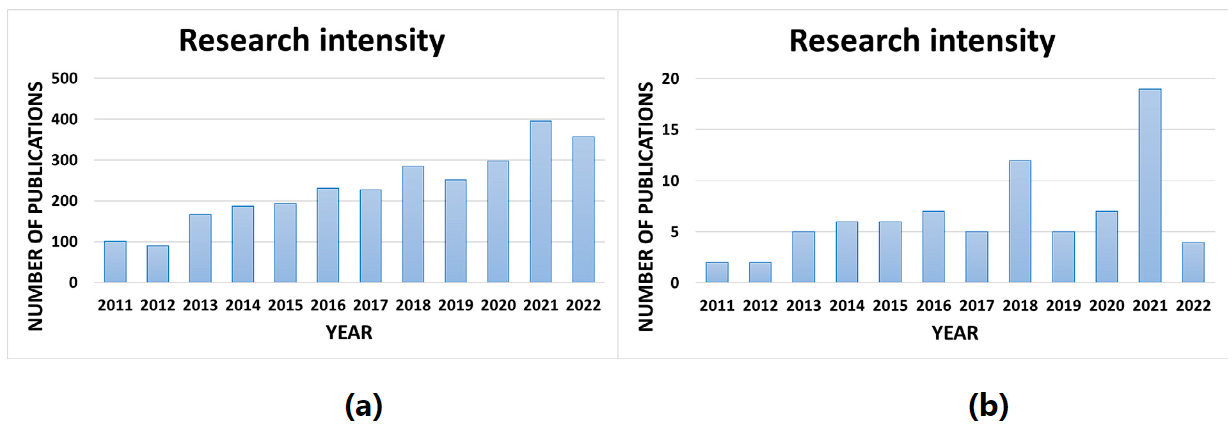
Figure 8a-b Research intensity on energy-driven biorefineries in the last 11 years. (a) Global (SCOPUS database search criteria: TITLE-ABS-KEY (biorefinery AND (biofuel OR (electricity OR heat OR power))) AND PUBYEAR > 2010 AND PUBYEAR < 2023 AND (LIMIT-TO (DOCTYPE, "ar"))); (b) Colombia (SCOPUS database search criteria: TITLE-ABS-KEY (biorefinery AND (biofuel OR (electricity OR heat OR power))) AND AFFIL (colombia)) AND PUBYEAR > 2010 AND PUBYEAR < 2023 AND (LIMIT-TO (DOCTYPE, "ar"))).
Table 2 shows the distribution of publications by region and department at the national level associated with the theme of energy-driven biorefineries, where there is a wide dominance of the coffee-growing region and very low production in the Pacific region. On the other hand, from the perspective of the most studied crops, Table 3 shows that the most studied ones are oil palm and sugarcane, which is related to the country's implementation of bioethanol (sugarcane) and biodiesel (oil palm) production as first-generation biofuels. It is worth noting that a large portion of the publications are related to the production of second-generation biofuels (from crop residues), as well as molecules/products of interest to the chemical industry.
Among the crops studied, sugarcane is the only one currently established in the biorefinery configuration. This is because it produces sugar, bioethanol (biofuel), electrical/thermal energy (internal consumption and sale to the National Interconnected System), and organic-mineral fertilizer. For this purpose, the sugar sector is made up of 15 cane processing plants (15 are cogenerators of energy, 8 produce only sugar, 6 produce sugar and ethanol, 1 produces only ethanol) that transformed more than 175,000 ha (127 tons of cane/ha) in 2021 distributed in the geographical Valley of the Cauca River (comprised of the departments of Valle del Cauca, Cauca, Risaralda, Caldas, Quindío, and Meta) 96-98.
Table 2 Distribution of publications related to energy-driven biorefinery at the national level by region and department (Source: own elaboration).
Region | Department | N° Publications |
---|---|---|
Caribbean | Bolívar | 12 |
Coffee Axis | Antioquia | 4 |
Caldas | 47 | |
Pacific | Nariño | 2 |
Valle del Cauca | 1 | |
Central East | Cundinamarca | 1 |
Santander | 11 | |
Bogotá | 2 | |
Total | 80 |
Table 3 Top 5 Most Investigated Crops in Biorefinery Configuration at the National Level (Source: Own elaboration)
Tipo de cultivo/residuo | N° Publicaciones |
---|---|
Oil Palm and Residues | 14 |
Sugarcane and Residues | 10 |
Coffee and Residues | 9 |
Banana/Plantain and Their Residues | 9 |
Microalgae | 9 |
Based on the above, 6 plants can be taken as the unit of analysis to address the real potential of carbon capture and storage (CCS) in the energy-driven sugarcane biorefinery. Below are the modules associated with bioenergy production:
Bioethanol Production
Close to 85% of the sugarcane crushed in Colombia is used to produce sugar and fuel alcohol (bioethanol), as part of a dual and successive process. In equivalent terms, approximately 20% of the sugarcane is allocated to alcohol production 99, aiming not to jeopardize food production for human consumption.
The scheme for ethanol production directly from sugarcane (1st Generation bioethanol) is shown in Ref. 58. In this process, sugarcane is harvested and crushed. The juice (rich in sugars) is conditioned to make it more susceptible to attack by microorganisms during fermentation. The cellular biomass must be separated from the fermented broth, followed by ethanol separation (distillation) and subsequent dehydration through different unit operations to obtain anhydrous ethanol. This process can also use sugarcane molasses, as well as other streams derived from the sugar extraction process in sugar mills. The theoretical stoichiometric yield for this process is 0.511 g of ethanol and 0.489 g of CO2 per 1 g of metabolized glucose. Considering the yeast Saccharomyces cerevisiae, experimental and industrial levels have been observed to achieve between 87% and 95% of the theoretical yield 100.
The byproducts of the industrial bioethanol production process include wastewater, vinasse, and CO2. While wastewater is treated through aerated surface basins (lagoons) before release, CO2 is vented into the atmosphere. On the other hand, vinasse is concentrated by removing water, yeast, and organic matter, which are then recirculated to the bioethanol fermentation reactor 93. According to Yáñez et al. 94, the theoretical estimate of CO2 generated in bioethanol production in a national-level plant corresponds to 333,060 tons of CO2 per year. This estimation was based on an emission factor of 0.968 tCO2 per ton of anhydrous ethanol produced, the annual ethanol production (397 million liters), and a capacity factor of 56%.
This CO2 value could change depending on the realization of research proposals aimed at producing second-generation bioethanol from some sugarcane residues, as well as from process byproducts, or making the production of first-generation bioethanol more efficient. This would allow for the integration of new process modules into the current biorefinery configuration.
Thus, the production of this biofuel in the country has been driven since 2004 by the bioethanol blending mandate (Decree 4892, Laws 788 and 939), in response to energy security concerns and the ambition to reduce emissions in the transportation sector. This mandate defined a 10% blend of bioethanol by volume (E10) to be used in gasoline fuel for road transport. The mandate is regulated by the Ministry of Mines and Energy and is accompanied by tax incentives for the sale of bioethanol and the importation of processing machinery 93. Additionally, Resolution 40177 of 2020 101 defined bioethanol as a low-emission fuel, placing it among the options for less polluting fuel. The participation of this biofuel in the country's energy transition process is also related to the National Energy Plan 2020-2050 102, which includes the sector in the modernization scenario, as well as the Indicative Action Plan of the Energy Rational Use Program (PAI-Proure) 2021-2030 103, which defines the roadmap for the use of biofuels in the transportation sector. With the current proposals, the aim is to reduce energy consumption in the transportation sector (which represented 41% of the country's energy in 2019) and dependence on fossil fuels (96% of energy is concentrated in this type of fuel) by 2050, impacting the GHG emissions contribution of this sector (which represented 38.6% of GHG emissions by the energy sector in 2018).
In figures, ethanol is the most consumed biofuel worldwide (representing 71.3% of the biofuels market) 104, and the market size is projected to grow from USD 33.7 billion in 2020 to USD 64.8 billion by 2025, at a compound annual growth rate (CAGR) of 14.0% from 2020 to 2025, attributed to the mandatory use of bioethanol fuel blends in several countries to reduce greenhouse gas (GHG) emissions and increase vehicle fuel efficiency 105. In Colombia, an expansion of bioethanol production is expected once clarity is provided on prices and market conditions (including imports from the United States) 96.
Cogeneration of energy (electricity and thermal energy production)
The most important residue derived from sugarcane processing is bagasse, and currently, processing plants generate between 6-7 million tons per year (106). 85% of this bagasse is mainly used for cogeneration of energy in the same mills, and the remaining 15% is used for the paper industry. Sugar mills cogenerate to meet their electricity needs and sell the surplus to the National Interconnected System (SIN). This bioelectricity is mainly produced during the dry season, complementing electricity from hydroelectric energy and thereby reducing the use of energy generation from petroleum derivatives on the margin 100-106. According to the Ministry of Mines and Energy (2021), cogeneration from sugarcane bagasse represents 0.8% of the national energy matrix, where it represents 20% for the industrial sector 103. In 2021, the installed cogeneration capacity reached 336.2 MW, of which 156.7 MW were allocated to placing surplus energy on the market. By 2024, it is expected that other capacity expansion projects for cogeneration will come into operation, increasing the total capacity to 392.4 MW, representing a 17% increase compared to the capacity in 2021 96. This is driven by national policies on renewable energy from non-conventional energy sources (NCES), aiming to achieve a 10-20% participation in the energy matrix by 2050 102.
In Colombia, cogeneration has been encouraged since the enactment of Law 788 of 2002, which creates an exemption from income tax on the sale of energy from biomass. Over time, this legal framework has been adjusted. For example, on July 16, 2008, Law 1215 came into effect, exempting cogenerators from paying the 20% contribution on the energy they generate for their own consumption. Continuing this progress, Resolution CREG 005 of February 2010 regulates cogeneration, distinguishing it from other types of generation, which allowed for incentives and specific conditions for cogeneration development. With the sanction of Law 1715 of 2014, which regulates the integration of non-conventional renewable energies into the national energy system, minimum incentive conditions are generated for investment in this type of energy 107. Finally, the recently issued Law 2099 of 2021 or Energy Transition Law strengthens the framework for energy generation from biomass 108, within which cogeneration is included. Additionally, the National Energy Plan 2020-2050 102 recognizes bioenergy as an element in the country's energy transition. Therefore, it is expected that the expectations of the sugar sector for cogeneration by 2024 can be realized.
Cogeneration is a process whereby electrical, mechanical, and thermal energy is simultaneously produced. In this sense, the energy in the form of heat produced by bagasse is used to generate steam, and then electrical energy is produced using turbo-generators, as shown in Ref. 58. However, the bagasse from milling (at a moisture content of 33% w/w) feeds the cogeneration system to supply heat to the process. Combustion is carried out with 50% excess air. This excess air increases energy efficiency by raising the temperature of the combustion gases. The heat obtained is mainly used for the production of high-pressure steam (HPS) superheated to 30 bar. In a second stage, low-pressure steam (LPS) saturated at 3 bar is produced. The hot temperature of the residual combustion gases partially dries the moist bagasse and preheats the fresh combustion air to the boiler. Energy integration minimizes energy consumption in the plant, increasing energy production. The HPS drives a steam turbine for power generation, while the LPS heats the ethanol distillation reboilers. The final combustion gas is at the appropriate temperature for release into the atmosphere, which is common practice for the industry 58. This is because cogeneration from sugarcane bagasse is a particular case regarding the accounting of externalities, as although biomass combustion generates CO2, it is considered part of the natural carbon cycle on Earth. Plants take CO2 from the air to grow and then return it to the air when burned, so they do not generate a net increase in CO2109. Therefore, there is a great opportunity to evaluate the CO2 capture potential of this process at the national level (BECCS case) to achieve negative CO2 emissions. This could be achieved either through a CO2 capture module after energy cogeneration (post-combustion) or before/during the energy cogeneration process (pre-combustion or oxy-combustion).
It is worth noting that the amount of CO2 currently emitted by sugarcane bagasse cogeneration processes will increase in the future due to ongoing capacity expansion projects in the sector 106. It is also necessary to mention that there is moderate research activity at the national level in this field, focusing on gasification and combustion processes aimed at achieving greater efficiency in utilizing sugarcane residues to produce bioenergy.
Regarding the identified opportunity, in Colombia, various CO2 capture technologies have been investigated at the laboratory scale for point sources of industrial emission from energy generation.Table 4 shows the distribution of publications by region.
It can be observed that research has mainly focused on adsorption technology, which can be attributed to the ease of synthesizing adsorbent materials, the wide variety of these materials, and the low complexity in setting up experimental adsorption tests in the laboratory. However, several of the materials proposed in this field are not viable for scaling up for use at the industrial level, such as for CO2 emissions from sugarcane biorefineries. Meanwhile, Table 4 shows that the Central-East region has conducted the most research on this topic, involving a greater number of research groups. Finally, the Caribbean and Pacific regions each have their own research focus, with one research group each. It is important to note that none of the proposals have been tested or simulated in gases resulting from bioenergy production.
Table 4 Number of publications related to the theme of CO 2 capture (CCS) for point sources of industrial emissions from energy generation at the national level by region and department (Source: Own elaboration)
Region | Department | Research group | CCS Thecnology | Number of Publications |
---|---|---|---|---|
Central-East | Bogotá | 5 | Adsorption | 39 |
Cundinamarca | 1 | Absorption | 2 | |
Absorption and Sieve | 1 | |||
Santander | 2 | Adsorption | 7 | |
Boyacá | 1 | Adsorption | 4 | |
Coffee Axis | Antioquia | 3 | Adsorption | 9 |
1 | Absorption | 2 | ||
Absorption or Oxi-combustion | 1 | |||
1 | Oxi-combustion | 1 | ||
Caldas | 1 | Absorption or Distilation | 1 | |
Risaralda | 1 | Adsorption | 1 | |
Caribbean | Bolívar | 1 | Membrane Separation | 4 |
Pacific | Valle del Cauca - Cali | 1 | Chemical Looping Processes (CLP) | 9 |
On the other hand, regarding Oxygen Carriers (OC), the group's research has focused on the development of low-cost OCs based on local manganese, iron, and copper minerals. In this regard, there is expertise in conditioning these types of materials, which is vital for the development of OCs from industrial by-products, such as those from the iron and steel production industry. It is worth noting that this represents an important opportunity within the framework of the country's circular economy, considering that the iron and steel production sector at the national level faces challenges in closing the cycles of several by-products (CONPES 3934), such as slag (approximately 296,099 tons were produced in 2018) and lamination husk or calamine (20 kg are produced per ton of steel), which, as mentioned in previous paragraphs at the international level, have been used as OCs 110.
Thus, there is only one national study at the simulation level focused on using the CO2 generated in a sugarcane biorefinery (fermentation and cogeneration) to cultivate microalgae for the purpose of obtaining 2nd and 3rd generation biofuels 98. However, the study uses only a fraction of the generated CO2 (limited by the microalgae cultivation process itself), and the rest is emitted into the environment, so it would not be considered complete CO2 capture. Similarly, it is indicated that the proposed CO2 capture technology in the simulation corresponds to absorption.
Conclusions
From the analysis of international information, the potential and relevance of using BECCS technologies in achieving global environmental objectives for reducing CO2 emissions into the atmosphere are evident. This is why BECCS technologies are included among the array of strategies promoted by environmental policies of major developed countries.
CO2 capture technology from thermal processes such as those carried out in the hydrocarbon industry is considered mature (amine adsorption) and could be applied in conjunction with bioenergy generation technologies. However, there are significant drawbacks to its implementation in biomass combustion, including a critical increase in water usage, toxicity of residues, and solvent degradation. Consequently, second-generation CO2 capture technologies in biomass energy cogeneration (bioelectricity and/or heat), such as processes with Chemical Looping Process (CLP), have begun to gain traction in academic circles.
From an implementation standpoint, it is noteworthy that before the deployment of BECCS technologies on a country-wide scale, it is advisable to conduct various technical-economic evaluations/simulations in large agro-industrial companies that could achieve high BECCS efficiencies (to achieve deep reductions in atmospheric CO2), such as energy-driven biorefineries that play a vital role in decarbonizing the energy matrix, such as those linked to sugarcane.
The implementation of BECCS technologies in sugarcane-linked biorefineries highlights their novelty in the theoretical and experimental estimation of the CO2 capture potential generated by CLP under plant parameters in pre-combustion and/or post-combustion configurations. Furthermore, within the framework of the circular economy, the opportunity to develop low-cost oxygen carriers from by-products of the iron and steel production industry is emphasized.
At the national level, the policy framework oriented towards the storage of CO2 captured from emission sources is relatively new. Therefore, theoretical-experimental research in this field at the national level by academic institutions that can inform technological plans or roadmaps by environmental control/execution agencies is scarce. Hence, the opportunity to generate knowledge around the behavior of different energy-driven biorefinery schemes at the local level, coupled with CO2 capture technologies (BECCS), as well as the technical conditions for the safe disposal of this through storage in different geological formations available, is highlighted.