Introduction
The processes controlling the alteration of primary minerals depend on the relative mobility of some major components (Anderson & Hawkes, 1958; Johnson et al., 1968; White et al., 2001; Anderson et al., 2002; White & Brantley, 2003; Wilson 2004; Meunier et al., 2007; White & Buss, 2014). Basically, the unique genetic relationship between the soil and the parent material through the profiles helps to understand the role of rock weathering and soil formation. The influence of the parent material mineral composition on the genesis of the soils is still a question to be explored, as widely shown in the traditional literature (Chesworth, 1973a). Therefore, the main source of the bases is related to in situ chemical weathering of local bedrocks (Munroe et al., 2007). Special importance has been given to the removal of alkali and alkaline earth elements (Na, Ca, Mgand K) during the continental weathering (Chesworth, 1973b), due to the alteration of clay minerals and a high removal of Na from dissolution of feldspars and plagioclases, followed by a progressive enrichment in silica and Al and Fe sesquioxides (Wilson, 2004); occasionalyy, desilification occurs at the final weathering stage. During weathering the rock forming primary minerals, micas and feldspars, are depleted in bases; in this way, K release is considered a good measure of the degree of alteration of the aluminium silicate minerals (Wilson, 2004). In addition, chemical weathering of silicate rocks through acid hydrolysis could take place and lead to an exchange of Na, K, Ca and Mg for H and, perhaps, a loss of Si (Bain et al., 1990; Ezzaim et al., 1999a, 1999b; Papoulis et al., 2004). According to Banfield & Eggleton (1990). Both processes, the loss of K and Na and the enrichment in Al, are in consonance with an advancing process of kaolinization and formation of iron and aluminium oxyhydroxides too. This fact has been explained by some authors for weathering soil profiles from the Iberian massif (Molina et al., 1990; Vicente et al., 1991; Vicente et al., 1997; Molina & Cantano, 2002; Jiménez-Espinosa et al., 2007; Núñez & Recio 2007; Fernández-Caliani & Cantano 2010; Doval et al., 2012).
Other relevant factors regarding weathering in the traditional literature include the fundamental alteration mechanisms and the products derived from the primary minerals (Banfield & Eggleton, 1990; Merriman et al., 1990; Robertson & Eggleton, 1991; Robert & Tessier, 1992; Gardner & Walsh, 1996; Aspandiar & Eggleton, 2002, Spears, 2016). Many secondary clays are commonly considered an alteration product in acidic soil environments as explained by Pédro (1997). For example, the evolution of parent rocks controlled by an hydrolysis phenomenon, including bisialitisation, monosiallitisation and allitisation, within the formation of minerals from the smectite group, kaolinite group gibbsite and iron oxides group found in the pedological horizons. Evaluating geochemical composition, not only releases basic information about the formation conditions, but also the evolution and the alteration stages of the rock substrates and soils. Wilson (2004) noted that the differences in alteration mechanisms, products and the rates of weathering commonly observed among bedrock and soil environments can differ widely, even in the same geological units. Nesbitt et al. (1980) already mentioned degradation and leaching dominate the early weathering stages, whereas during the advanced stages, ion exchange and adsorption onto clays are of most influence. Banfield & Eggleton (1990), Fedo et al. (1995) and Price & Velbel (2003) suggested a sequential formation of secondary weathering products and pointed out the utility of evaluating weathering indices attending to simple profile depth variations. Thus, evaluating chemical weathering indices and element relations between mobile or immobile elements help rapidly to understand weathering intensity, possible processes and, sometimes, soil grade of development and evolutionas proven for instance by Gardner & Walsh (1996). The main chemical alteration indices (Ruxton 1968; Parker 1970; Nesbitt and Young 1982; Harnois 1988; Jayawardena and Izawa 1994; Fedo et al. 1995) have deeply contributed to the understanding of weathering a great variety of geological substrates. Even though, some of the conventional indices, mainly use to estimate weathering intensity, should be reformulated as an alternative approach to evaluate different rock weathering grades (Guan et al., 2001; Meunier et al., 2013). Nevertheless, chemical indices of alteration resulted really helpful when evaluating the losses or enrichment of base cations from the rocks However, evaluating the release of silica and relative accumulation of iron and aluminum in soils from the rock weathering requires being adapted (Meunier et al., 2013).
Additionally, several studies highlighted implications for paleoweathering conditions and provenance as pointed out by Fedo et al. (1995). Recent reviews on climatic and geochemistry (Perri, 2020 or Dinis et al., 2020) enlighten their strongly interactions using chemical indices as proxies. Recently, Dinis et al. (2020) have recognised these significant relationships between mineralogical and geochemical signatures of sediments and rainfall registers at catchment scale. Moreover, some other factors take an important role in such complex context, particularly diverse physical factors driven by topography as suggested by Tardy et al. (1973).
This work characterizes the mineralogical and geochemical composition of a series of six natural profiles consisting of soils (S series) and parent rocks (R series) developed on Palaeozoic lithologies from the Iberian Massif under similar weathering conditions in temperate and acidic environments. The influence of lithology on mineral and geochemical properties and the assumption of mineral alteration by differential weathering have been addressed. The specific aims of this research are: 1) characterize the major compositional mineralogy, particularly focusing on fine-grained and clay fractions in six selected weathering profiles; 2) identify the geochemical signatures according to the chemical indices of alteration; 3) understand the factors controlling the weathering processes and 4) explain possible differential chemical weathering mechanisms presumably taking place in such acidic environments.
Geological setting
The profile sites studied are located in the NW part of the Iberian Massif (Variscan Orogenic Belt) included in the West Asturian-Leonese Zone (WALZ) (Figure 1), specifically in the Navia-Alto Sil Domain (NASD) (Pérez-Estaún et al., 2004; Marcos et al., 2004). The Variscan Orogeny in the WALZ was a polyphasic deformative process associated with synkinematic metamorphism under epizonal deformation conditions, mainly to greenschist facies (Martínez-Catalán et al., 1990; Suárez et al., 1990), with mineral paragenesis associations typical of the chlorite zone. The WALZ, including the Lower to Upper Paleozoic units, is characterized principally by the presence of a thick assemblage of Cambrian-Ordovician siliciclastic rocks, which shows an overall structure, formed largely by recumbent folds and thrusts.
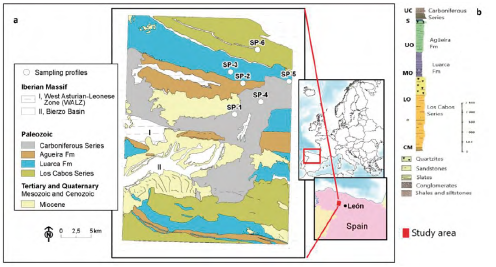
Map source: The National Geological Survey Institute of Spain (IGME) modified from Rodríguez Fernández (1982).
Figure 1 Weathering sampling profiles (SP-1, SP-2, SP-3, SP-4, SP-5, SP-6) a) location in the lithological units from the WALZ (NW Iberian Massif, Spain). b) Synthetic stratigraphic column modified from Pérez-Estaún (1990) showing formations and series form ages UC: Upper Carboniferous; S: Silurian; UO: Upper Ordovician; MO: Middle Ordovician; LO: Lower Ordovician and CM: Cambrian.
The lithostratigraphic sequence starts with the Lower Paleozoic materials of Cambrian to Ordovician age (Pérez-Estaún et al., 1990). It begins with detritic facies characterized by an alternation of quartzites with slates, siltstones and sandstones referred to as the Los Cabos Series (Cua). A range of later Paleozoic materials, referred to as the Transition Layers, gradually gives way to the Luarca Formation (Lua), an assemblage of fine grained black slates, rich in sulphides like pyrite and organic matter. This is immediately followed by the Agüeira Formation (Agu), formed by alternating sandstones, siltstones and shales with a presence of turbidite depositional environment, which sometimes culminates in a quartzite layer. The Silurian strata, poorly represented, are found in a gradually alternating section, including fully sandy phases with varying proportions of slates. These are discordantly overlain by Upper Carboniferous (Carb) (Stephanian B-C) synorogenic materials of the El Bierzo coal basin (Barba et al., 1994), which represent the development of a strong paleorelief. The Carboniferous unit consists of a broad range of sediments such as alternating layers of sandstones, shales, siltstones and conglomerates combined with intermediate coalbed deposits (Colmenero et al., 2002; Wagner, 2004).
Materials and methods
The sampling profiles (SP) are located in six different lithostratigraphic units within the Paleozoic succession named as Cua, Lua, Agu and Carb (Table 1): Los Cabos Series (SP-6)-Cua, Luarca Formation (SP-3 and SP-5)-Lua, Agüeira Fm (SP-2)-Agu, and Carboniferous Series (SP-1 and SP-4)-Carb. Samples including partially weathered or fresh rocks (R-series) were named as: R1 (Figure 2a), R2 (Figure 2e) , R3 (Figure 2b), R4 (Figure 2f), R5 (Figure 2g) and R6 (Figure 2h); other altered weathered geological materials in contact with the bedrocks beneath, including the soil (S-series) named as: S1 (Figure 2a), S2.1 and S2.2 (Figure 2e), S3.1 and S3.2 (Figure 2b), S4 (Figure 2f), S5 (Figure 2g), S6 (Figure 2h), were collected in the sampling sites from SP-1 to SP-6 as showed in Figure 1.The correspondence between the profile sites and samples series is specified below (Table 1): SP-1 (samples R1 and S1)-Carb; SP-2 (samples R2, S2.1 and S2.2)-Agu; SP-3 (samples R3, S3.1 and S3.2)-Lua, SP-4 (samples R4 and S4)-Carb; SP-5 (R5 and S5)-Lua; SP-6 (R6 and S6)-Cua.
Table 1 Descriptive features of the investigated sampling profiles soil horizons (S-series) and bedrocks samples (R-series) location (UL: Upper and LL: Lower Level of weathering) according to depth, general profile weathering degree descriptive material features (HD: high; MD: moderate, LD: low degree), lithological units, parent rocks and soil series. *Acronym for the geological unit's name
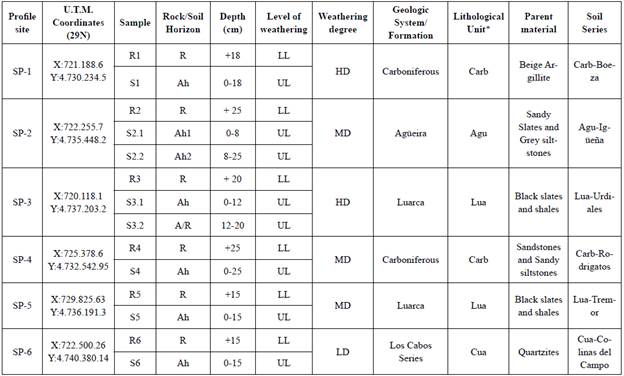
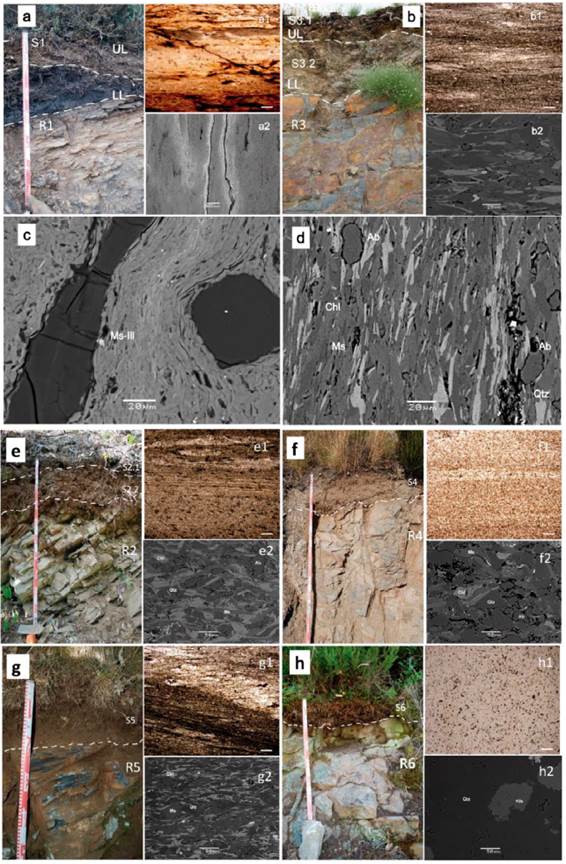
Figure 2 Soil profiles showing two different levels of weathering UL: upper level; LL: lower level where samples were taken: image a) profile SP1 (S1 and R1) and image b) profile SP3 (S 3.1, S3.2 and R3). Images from e) to h) soil profile sites SP2, SP4, SP% and SP6 respectively. Thin sections showing the rock samples microtextures and granulometry R1 (image a1) and R3 (image b1); R2 (image e1), R4 (image f1), R5 (image g1) and R6 (image h1). Observational scale at 1 mm. SEM BSE images (a2 at 100 Lim and b2 at 20 um captured scale; e2, f2, g2 and h2 at 50 um captured scale) SEM BSE detailed images for identification of muscovite (Ms) and illite (Ill) on R1 (image c) and quartz (Qtz), muscovite (Ms), chlorite (Chl) and albite (Ab) on R3 (image d).
Microscopy
Scanning electron microscopy with energy dispersive X-ray spectrometry (SEM-EDS) was carried out as a supporting tool to validate mineral petrographic analysis. Thin sections from sample substrates were previously studied by optical microscopy (POM, transmitted and reflected light), using a Nikon Eclipse E600 POL petrographic microscope. The same polished thin sections were subsequently carbon coated and studied under a JEOL JSM-6480 high resolution scanning electron microscope (SEM), equipped with an Oxford Instruments D6679 Energy Dispersive X-ray Spectrometry (EDS) detector. For each specimen acceleration voltages range of 10 to 20 keV, working distance was 10 mm and count rate set at 15000 counts/s. Back-scattered electron (BSE) images were acquired and processed using INCA Energy Software provided by Oxford Instruments.
X ray diffraction
Representative portions of powdered rock samples were subjected to X-ray diffractometric analyses. Air dried rock samples were pulverized in a Restch PM400 automatic rotary planetary mortar mill, collecting the fraction <125 µm. XRD analyses were performed at room temperature and 400 C during 0.75 hours. Representative samples were subjected to X-ray powder diffraction, using a SIEMENS Bruker D5000 series diffractometer system equipped with Cu-K-alpha radiation at 40kV and 30 mA, 0.2 mm receiving slit, a range of 2°-58° 2θ and graphite secondary monochromator facilities. Scanning step size was set at 0.02 ° 2θ and the scanning rate 1 second per step, giving a scan speed of approximately 2° 2θ per minute and a total duration of 0.75 h.
Oriented aggregates of the <2.0 um fine clay fraction were obtained from the soil samples, using suspension after hexametaphosphate dispersion, separation by sedimentation and settling techniques after 24 hours (Klug & Alexander, 1974). The clay samples were dried at 40°C, homogenized and manually powdered using an agate pestle and mortar. Previously, the sample pretreatment was the organic matter removal with a hydrogen peroxide agent (30% v/v) at 25 C. Duplicate samples were mixed and shaken in a tapered centrifuge tube. The remainder, which stayed in suspension, was removed and dropped on to a circular glass slide. This was then allowed to dry in the laboratory atmosphere, leaving an oriented clay film on the surface of the glass slide. Each glass slide, with its clay film, was subjected to X-ray diffraction analysis using a Panalytical Empyrean II diffractometer in the air-dried state, using Cu-K-alpha radiation and in this case a range of 2°-46° 2θ for soil clay samples. The slides were then placed in a desiccator containing ethylene glycol, and exposed to the glycol vapour at room temperature for several days. The glycol-treated slides were then heated at 400 C for one hour and subjected to XRD analysis. Identification of the minerals in the parent rocks and soil clays was carried out following the general indications of Moore & Reynolds (1989).
Chemical analyses
Chemical analyses were performed within duplicated samples including bulk chemistry and fine grained fractions <2.0 um for clay soil samples. Major element analyses were carried out by X ray fluorescence spectrometry (XRF) and, alternatively, some of the major and minor elements were measured by Inductively Coupled Plasma Mass Spectrometry (ICP-MS). The fused borosilicate disks were prepared with 95%Pt/5%Au crucibles, adding 0.500 g of a 1:1 mixed fusion flux of Lithium tetraborate/metaborate (50% Li2B4O7/50% LiBO2), in a fusion melting induction furnace heated to 1000 °C during 20 min, using a non-wetting agent of LiBr. They were analysed in a BRUKER-NONIUS S4 Pioneer wavelength dispersive XRF spectrometer. The samples prepared following the specifications of the alkali fusion technique required acid dissolution with HNO3 5% (v/v). They were measured by ICP-MS in a Thermo Scientific quadrupole XSeries2, with detection limits of 0.01% using as standard elements In, Sc and Rh. The major element oxides CaO, MgO, Na2O, K2O, SiO2, Fe2O3, Al2O3, P2O5, MnO and TiO2 were expressed as weight percentages (wt °/o). Previously, representative splits of each sample were oven-dried, and then heated at 975 C for 12h in a high temperature furnace HOBERSAL12-PR400to obtain the Loss on Ignition (LOI) percentage in each case.
Results
Mineralogy
In the preliminary mineralogical identification for rocks the principal minerals are phyllosilicates of the mica group, mostly Ms-Ill (Figure 2c) and chlorites (Figure 2d) respectively, accompanied by quartz (3.34 Å) and feldspars, according to the major peaks (3.19 Å and 3.22-3.24 Å) correspond to plagioclases (albite) as confirmed by SEM analyses (Figure 2d) or K-feldspars, in the case of R6 (Figure 2h2). The microscopic and SEM BSE analyses can be found detailed on Figure 2. It can be noted that albite is present on R3, R5 and R4 (Figures b2, f2 and g2) and, clearly more abundant, on R2 (Figure e2), all assessing the XRD analyses too.
In addition, kaolinite (Kln) is only present in some of the R-samples (Table 2). There is a relative higher abundance of chlorite in samples R3, R5 and R2, the albite content is higher in R2, however kaolinite content is higher in R1 (Table 2). The major minerals in the sample R1 are mica (muscoviteillite), quartz and kaolinite respectively (Ms-Ill, Qtz and Kln) as seen in Figure 3. Kaolinite is clearly identified in sample R1 (Figure 3a). By contrast, an iron-rich chlorite identified in samples R2 to R5 as shown in (Figure3b), SEM analysis confirm that correspond to chamosite (Figure 3b). From samples R2 to R6 the peaks at 10 Å and 5.0 Å in all patterns analysed are indicating mica, but finally the SEM results shown enough evidence to be identified as muscovite, so it was also confirmed by SEM. It is noted that the peak of 3.34 Å can be also of mica-illite, so the reflection of 4.26 Å would be enlightening. This also applies to sample R1 (Figure3a), although overall intensity is relatively low for that pattern, suggesting a mica (muscovite) rather than a less well-ordered illite phase as confirmed by SEM analysis (Figure 2c).
Table 2 Mineral identification in the rock samples and relative mineral abundance referred to the total mean quartz contents. Abbreviations: Qtz = quartz; Ms = muscovite; Ill = illlite; Chl = chlorite; Kln = kaolinite; Pl = plagioclases; Ab = albite; KFs = K-feldspars. Conversion scaled weights: ****= 3.0 (very abundant) ***= 1.0 (abundant) **= 0.4-0.6 (frequent) *= 0.3-0.2 (present) tr <0.2 (traces) and - = not identified.
Profile site | Sample | Qtz | Ms | Ill | Chl | Kln | Pl | KFs |
---|---|---|---|---|---|---|---|---|
SP-1 | R1 | ** | *** | *** | - | ** | - | - |
SP-2 | R2 | ** | *** | ** | - | *** | - | |
SP-3 | R3 | ** | *** | ** | * | * | - | |
SP-4 | R4 | *** | ** | * | - | ** | - | |
SP-5 | R5 | ** | *** | ** | * | * | - | |
SP-6 | R6 | **** | tr | - | - | tr | tr |
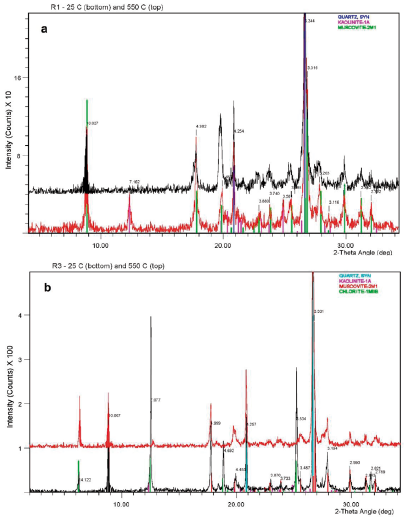
Figure 3 Rock powder unoriented XRD pattern, air-dried and solvated at room temperature 25 C (bottom line) and heated at 550 C (top line). Major minerals identified in a) sample R1 and b) sample R3. Only the range from 5° to 35°- 29 is shown in the pattern.
The principal mineral phases present in the soil samples from the clay oriented aggregates patterns are given below in Table 3. In particular, the sequences are essentially the same identified before (micaceous phases and illite, with iron rich chlorite, traces of quartz, feldspars and minor kaolinite with some accompanying iron oxides). Particularly, only minor ferric amorphous species like lepidocrocite and iron oxides were identified as traces (Figure 4a). A Small peak at 6.2 Å on glycol (and also air-dried diffractogram) that disappears on heating suggests a trace of iron oxides corresponding to lepidocrocite in S5 and S1 (Figure 4a). The presence of ferroan chlorite has been confirmed in samples S2.1, S2.2, S3.1, S3.2 (Figure 4b) and S5 through the strong peaks at 7.1 Å and 3.53 Å, with smaller peaks at 14 Å and 4.7 Å, suggesting an iron rich variety. Small peak at 3.58 Å on saturated patterns indicates additional minor kaolinite detected in samples S3.1, S3.2 (Figure 4b), S4 and S5. Peak at 7.1 Å, not significantly affected by heating accompanied by a small peak at 3.58 Å, especially on heating, suggests that kaolinite is also present (Figure 3a). The absence of strong peaks at 14 Å, 7.1 Å and 3.53 Å, especially with glycol, indicate that chlorite is not present in this particular sample (Figure 4a). Moreover, in the samples from S2 to S5 peaks at 14 Å, 7.1 Å and 3.53 Å, glycol solvated (Figure 4b), indicate presence of chlorite; a small peak at 3.58 Å on heating, suggests that kaolinite is also present (Figure 4b). Quartz (peaks at 3.35 and 4.26 Å) and K-feldspar (peak at 3.2 Å) are also representative in the composition of the soil samples showing sharp peaks in reflections (Figure 4b).
Table 3 Total chemical composition determined in the six profiles for parent rocks and soil samples. Contents of major components analysed by XRF and ICP-MS (weight %), *LOI (Loss On Ignition).
Profile site | Sample | Depth | SiO2 | Al2O3 | Fe2O3 | K2O | MgO | CaO | Na2O | TiO2 | P2O5 | MnO | LOI* | Total |
---|---|---|---|---|---|---|---|---|---|---|---|---|---|---|
SP-1 | R1 | +18 | 56.67 | 23.38 | 5.79 | 4.89 | 0.73 | 0.26 | 0.50 | 1.00 | 0.06 | 0.02 | 6.74 | 100.04 |
S1 | 0-18 | 67.01 | 17.58 | 7.31 | 3.85 | 0.63 | 0.41 | 0.48 | 0.79 | 0.16 | 0.02 | 1.15 | 99.39 | |
SP-2 | R2 | + 25 | 66.99 | 15.69 | 5.42 | 2.45 | 1.93 | 0.42 | 2.17 | 0.99 | 0.02 | 0.05 | 4.36 | 100.49 |
S2.1 | 0-8 | 73.23 | 13.38 | 5.75 | 2.58 | 1.35 | 0.47 | 0.84 | 1.62 | 0.08 | 0.04 | 0.85 | 100.19 | |
S2.2 | 8-25 | 68.46 | 15.30 | 6.40 | 2.98 | 1.64 | 0.42 | 0.88 | 2.09 | 0.08 | 0.04 | 1.11 | 99.40 | |
SP-3 | R3 | + 20 | 59.69 | 20.03 | 6.64 | 3.13 | 1.69 | 0.52 | 1.42 | 1.10 | 0.07 | 0.07 | 5.68 | 100.04 |
S3.1 | 0-12 | 63.84 | 19.85 | 7.91 | 2.97 | 1.35 | 0.35 | 0.80 | 1.14 | 0.12 | 0.04 | 1.04 | 99.41 | |
S3.2 | 12-20 | 65.22 | 19.82 | 7.50 | 2.15 | 1.53 | 0.34 | 0.70 | 1.24 | 0.10 | 0.05 | 0.76 | 99.41 | |
SP-4 | R4 | +25 | 65.14 | 18.17 | 3.86 | 3.40 | 1.50 | 0.34 | 0.91 | 1.09 | 0.06 | 0.03 | 5.63 | 100.13 |
S4 | 0-25 | 82.11 | 9.92 | 3.04 | 1.73 | 0.49 | 0.23 | 0.34 | 0.54 | 0.06 | 0.01 | 0.94 | 99.41 | |
SP-5 | R5 | +15 | 56.80 | 22.72 | 7.63 | 2.95 | 1.77 | 0.46 | 0.93 | 1.13 | 0.08 | 0.07 | 5.84 | 100.38 |
S5 | 0-15 | 60.80 | 22.11 | 9.30 | 1.94 | 1.06 | 0.32 | 0.86 | 1.61 | 0.15 | 0.17 | 1.07 | 99.39 | |
SP-6 | R6 | +15 | 97.45 | 1.07 | 0.17 | 0.44 | 0.05 | 0.42 | 0.00 | 0.08 | 0.02 | 0.00 | 1.01 | 100.71 |
S6 | 0-15 | 95.03 | 1.63 | 0.59 | 0.55 | 0.06 | 0.19 | 0.05 | 0.45 | 0.01 | 0.00 | 1.42 | 99.98 |
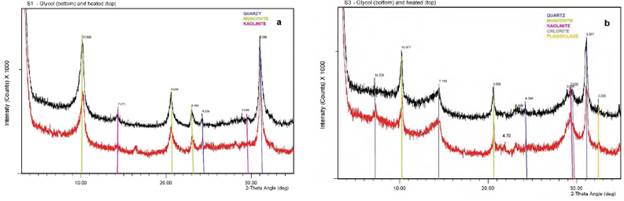
Figure 4 Clay oriented aggregates a XRD pattern from a representative sample a) S1 in the profile SP-1 and b) S3 in the profile SP-3 showing K-glycol treatment (bottom line) and heated at 400 C (top line). Only the range from 5° to 35°- 29 is shown to clarify the interpretation in the pattern.
Rocks and soils geochemistry
The contents of major elements in the samples are summarized in Table 3. The contents found in the soils are coherent with those ones present in the rocks through the soil weathering profiles. SiO2, Fe2O3 and Al2O3 contents are quite similar in soil profiles SP1 to SP5 being the most abundant elements. However in S6 from SP-6 the content of SiO2 reaches about 95%, while in the rest of soil samples get around 65-70%. The bases K2O, MgO, Na2O and CaO are generally lower than those present in the rocks. K2O (2-3%) shows higher contents among the last ones and, together with MgO (1-2%), are more abundant than CaO (0.2-0.4%) and Na2O (0.5-0.9%). The content of Na2O is slightly higher in the soil samples of SP-2 and SP-3 (Table 3). Regarding LOI results, the highest values correspond to SP-1 where R1 reaches 6.74 in the carboniferous series and the lowest to SP-6, where R6 is 1.01 (Table 3). The edaphic contents are little higher than the lithogenic ones for SiO2, Fe2O3 and Al2O3 (Table 3). The parent rocks show relatively important amounts of Na2O, Fe2O3 and MgO as well as K2O which are retained in the less altered minerals (Table 3). So, the contents found in the residual soils show an enrichment of SiO2, Fe2O3 and Al2O3 too (Table 3).
Chemical indices of alteration and element ratios
Weathering indices were calculated using molar proportions, increasing alteration indicated by increasing CIA (Chemical Index of Alteration; Nesbitt and Young, 1982), CIW (Chemical Index of Weathering; Harnois, 1988) and PIA (Plagioclase Index of Alteration; Fedo et al., 1995). CIW, CIA and PIA have reached higher values in SP-1, SP-3 and SP-5, all are in consonance with the geochemical base mobilization and depletion of major cations calculated (Table 4). However, minor differences have been shown in the present data from the soils and underlying rocks (Table 4). CIW and PIA show quite good correlation fitting (R2=0.49) (Figure 5a), although CIA and PIA reaches a better correlation R2=0.94. The fitting between CIW and CIA reaches a positive R2=0.99. The higher values of the CIA and CIW are indicating a possible high grade of alteration, mainly in the rock samples from profiles SP-1, SP-3 and SP-5. PIW present high values specifically suggesting one of the most possible ways of primary mineral alteration (Table 4). It is also assumed that LOI, the only one not expressed as a ratio, increases with the degree of weathering. LOI has also resulted higher in SP-1, which is in consonance with the rest of indices calculated. A R2=0.30 fitting in the correlation with CIW (Figure 5b) showing higher correlation in the rock series than the soil ones.
Table 4 Indices associated to the intensity of mineral alteration and weathering. Abbreviations: CIA: Chemical Index of Alteration; CIW: Chemical Index of Weathering; PIA: Plagioclase Alteration Index; Compositional molar ratios calculated from major oxides related to chemical and mineral weathering indices. Abbreviations: R2O3=(Fe2O3+Al2O3); BxO=(Na2O+K2O+CaO+MgO).
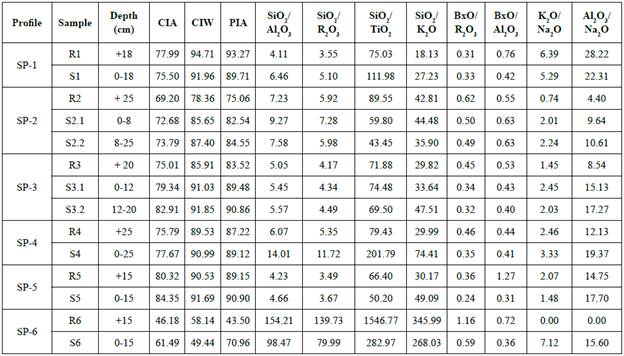
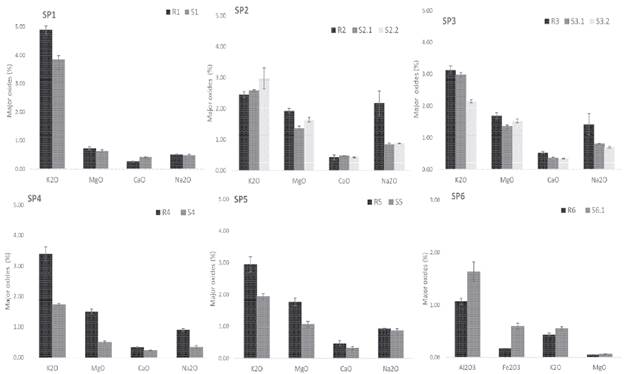
Figure 6 Enrichment and depletion in the soil profiles: composition of soil horizons showing mean concentrations and standard deviation of major components e xpressed in oxides (%).
Selected molar ratios, based on the principle that the ratio between concentrations of mobile (such as SiO2, CaO, MgO, K2O and Na2O) and immobile elements (Al2O3, Fe2O3, TiO2), should increase with weathering have been studied. These molar ratios such as SiO2/Al2O3 (Table 4) related to silica leaching result a dominant factor in the characterization of the weathering together with Chemical Weathering Indices (CIW, CIA and PIA) related to weathering intensity. The main chemical indices are expected to increase over time as leaching progresses. Some other selected ratios form the literature present a different behavior. For instance, SiO2/K2O tends to show lower values when the weathering is higher (SP-1) (Table 4). Opposite to that, SiO2/TiO2 remains almost constant with no noticeable differences as well as SiO2/Al2O3 and SiOVR2O3 both are in consonance among the rocks and soil samples not showing remarkable differences (Table 4). Other ratios regarding the bases give an estimation of weatherable primary minerals remaining in soils, such as BxO/ R2O3 and BxO/AbO3 (Table 4), decreases over time, so less weathered materials, for instance R6, present higher values (Table 4). KO/Na2O and AbO3/Na2O are both good indicators the weathering degree showing lower values related to less alteration (SP-2) and higher values for more altered phases (SP-1) (Table 4).
Discussion
Geochemical relations, elements mobility and mineral alteration
In general, the relative chemical composition variations are neither high nor too significant, comparing the upper and lower parts in the profiles, since the sampling depth is rather limited (Table 3). Generally, the profiles present an increasing content of some elements, noticeable in SiO2, Al2O3, and Fe2O3, from the parent rocks to the upper levels of the soil profiles (Table 3) while cations are leached. It is particularly important the relatively high K2O content found in all of soils, especially in SP-1 (Figure 6). Na2O, MgO and CaO are present in soils, but not significantly enriched (Figure 6). According to the results, there is a net gain of SiO2, AbO3, and Fe2O3 in the soils, in particular, from profiles SP-1, SP-3 and SP-5 (Table 3). Otherwise, K2O, MgO, CaO and Na2O are still relatively higher in the parent rocks (Figure 6), this last one especially in sample R2 from SP-2 due to the abundance of albite (Table 2). However, other leachable elements such as CaO do not show this clear tendency, so the content is rather limited in both rocks and soils, since it is only enriched in SP-1 (Figure 6). Meanwhile, soil MgO gain seems to be more evident, in lower parts of SP-2 and SP-3 (Figure 6) but limited in the rest. The K2O depletion from rocks and enrichment in soils, particularly shown in SP-1 and SP-6, is mainly the result of micas alteration (Figure 6).
The bases mobilities against iron sesquioxides are evident, but not high. The alteration causes hardly any variation in the relative amounts of Na2O and MgO, but leaching in the upper layers preferentially eliminates MgO (Figure 6). Exactly the same happens with CaO with respect to Na2O. The mobility of K2O is always greater than Na2O, and K2O, seems to be the most mobile, as there may be no minerals to retain it. In short, a gradual depletion of bases and enrichment in SiO2 and iron and aluminium sesquioxides is observed. Al2O3 contents found in soils are not higher than those ones in rocks (Figure 6). Al2O3 is slightly less common in soils, particularly in SP-4 and SP-1 and less noticeable in the rest (Figure 6). Indeed, the enrichment of silica, though not marked, is observed in most of soil profiles, especially in SP-4. SiO2 accumulation is normally followed by SiO2 mobilization and partial desilification, according to the removal as soluble colloidal form leached out of the profile. Under these climatological conditions the intense leaching left a material poor in bases, since there is hardly any variation in their content.
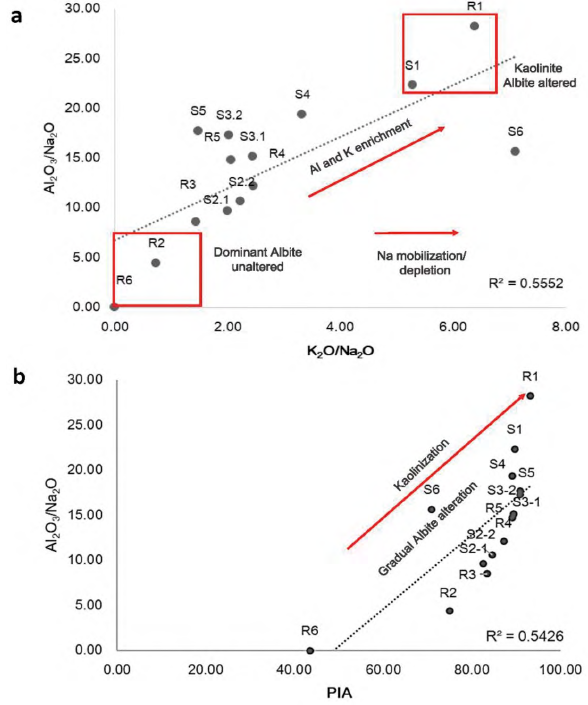
Figure 7 Cross data plots within linear correlation and R2 fitting for a) Al2O3/Na2O and K2O/Na2O and b) Al2O3/Na2O and PIA
Lower Al2O3 found in soils than in underlying rocks must be related with other physical processes besides chemical decomposition related to the removal of fine-grained clay particles enriched in Al2O3. Dinis & Oliveira (2016) explained that the composition of metasedimentary-derived sediments is not substantially affected by their grain size distributions. It could be explained by the dissolution leaching out of the profile, as colloidal particles clay fractions enriched in Al2O3, as happens when SiO2 migrates in the edaphic environment. This could explain the lower Al2O3 contents found in some soils due to dissolution, leaching and final depletion out from the soil profile.
As seen before, the profiles experience a gradual and little noticeable mobilization of bases from the destruction of the dominant primary minerals, mainly micas, Fe-bearing chlorites and feldspars and plagioclases, which is followed by a depletion in the upper parts of the soil profiles as well, as seen in Doval et al. (2012), Ling et al. (2016) and (2018). These losses were particularly observed in the early stages of the soils pedological development in agreement with Senol et al. (2016). It is common generalizing the genesis of highly weathered soils which results in intense loss of bases (Na2O, CaO, K2O and MgO), whereas less soluble Al2O3 accumulates in newly formed solid phases such as secondary 1:1 phyllosilicates like kaolinite, or mineral forms of aluminium hydroxide, such as gibbsite too (Romero et al. 1992). However, Taboada & Garcia (1999) showed that much evolution of the material is not necessary to explain the abundance of kaolin minerals and gibbsite, as these are compatible in many saprolites with absolutely fresh feldspars; in this case explaining the coexistence of albite and kaolinite supporting the evidence shown in this paper. Taboada & Garcia (1999) also reported that gibbsite may even be the mineralogical product formed from the plagioclases in the initial weathering stages when intense leaching occurs.
Albite alteration supported by chemical indices
Indices associated to the intensity of mineral alteration and chemical weathering (CIW, CIA and PIA) give a quantitative measure of feldspar weathering, by relating Al2O3 enrichment in the weathering in contrast to Na2O, CaO and K2O, which should be removed from the soil profile during plagioclase or K-feldspar weathering (Nesbitt and Young, 1982; White et al., 2001; Price and Velbel, 2003). Some element ratios biplots shown in Figure 7, help to clarify the interpretation. The molar ratios Al2O3/Na2O and K2O/ Na2O (Figure 7a) show clearly differences, mainly in SP-2 sample R2 where albite composition is higher and the ratios for mobile/mobile elements K2O/ Na2O and Al2O3/Na2O (Figure 7a) show the opposite in SP-1 where kaolinite is dominant in composition, due to in R1 and S1 albite is not present. Al2O3/ Na2O with KO/CaO with a correlation fit R2=0.56, indicative of the possible weathering increase with a linear positive tendency of the plot (Figure 7a). Al2O3 and K2O enrichment and Na2O mobilization are both indicative of the gradual plagioclase alteration, so chemical indices support the main hypothesis in this case of albite alteration. The cross-dot plots reflect a good fitting and indicate mineral composition, where albite unaltered is dominant or kaolinite is dominant (Figure 7a). Furthermore, Al2O3/Na2O result a key factor in the characterization of the grade of weathering (Figure 7b), when plotting against Plagioclase Index of Alteration (PIA) (Fedo et al., 1995) and with Chemical Weathering Indices (CIW) (Harnois, 1988). Al2O3/Na2O is considered a good indicator of the degree of weathering if we supposed lower values for less altered materials where plagioclases (albite) still remain in parent rocks. Weathering chemical indices selected PIA and CIW show a clear correlation, similar to the one obtained for Al2O3/Na2O and PIA for rock and soil series (Figure 7b). This positive correlation (R2=0.54) indicates a progressive weathering of albite while advancing the kaolinization in the most altered soil profiles SP-1, as the higher PIA values suggest, and less alteration in SP-2 (Figure 7b). In short, cations such as Na, K and Ca commonly generated by the weathering of feldspar and plagioclases into kaolinite, are rapidly release in the soil domain (Fedo et al., 1995). PIA, CIW and CIA and other chemical relations such as Al2O3/Na2O help to understand the most reliable assumption that kaolinite comes from the gradual alteration of albite. Despite the evidence of element leaching, although quite limited, during the data evaluation, geochemical indices such as PIA and AbO3/Na2O ratio are good indicators of possible differential weathering intensities in these profiles.
Differential weathering signatures
The main findings point out some possible differential weathering signatures supporting the mineral alteration of albite, especially the clue given by the identification of primary phyllosilicates and their coexistence with other secondary phases. This finding gives some enlightening data indicative of possible different processes affecting the alteration profiles. Particularly, special emphasis is given to the albite occurrence, which is found coexisting with kaolinite in the mineral sequences found in the profiles. This assumption may be explained by at least three particular conditions as shown in literature. Kaolinite, firstly supposed to be directly inherited in soils from the parent substrates when formerly present (especially abundant in R1 from SP-1). However, this explanation was not enough in the profiles where kaolinite does not exist originally since the edaphic formation of this mineral from the weathering of silicates requires extreme alteration condition (Molina et al., 1990; Hernando Massanet et al., 2004); not possible in the current studied soil conditions. Secondly, the weathered plagioclases drive to the occurrence of derived secondary kaolinite associated to a pedogenic origin (Driese et al., 2007; Churchman & Lowe, 2012). Strong pedogenic dissolution and alteration of feldspars and mafic minerals to kaolinite, gibbsite and iron oxides, as well as geochemical losses of Na, Ca, Mg and K (Driese et al., 2007) must be necessary to understand this fact occurring under particular conditions. In this work, the results have shown certain amounts of amorphous species like lepidocrocite and other iron oxides which are also associated to organic phases and weathering process in slates and shales rich in sulphides and organic phases, which are the dominant parent rocks. Adding to the previous, the hydrolytic weathering the main cause of a progressive transformation of affected components into clay minerals, ultimately into kaolinite (Romero et al. 1992; Taboada & Garcia, 1999), following the silicate weathering generally known as monosialitization. This acid hydrolysis favors the mobilizations of some bases, including in later stages, and the release of Ba and K from the partial alteration of K-feldspars, plagioclases and micas (Fernández-Caliani & Cantano, 2010). In short, kaolinite has been widely shown as the stable weathering product of the feldspars, plagioclases (albite) and muscovite (Núñez & Recio, 2007). In such context, it could enhance to explain, at least partially, the formation of secondary clay minerals belonging to the kaolinite group and the expansive clays and 2:1 mixed layers, more abundant in areas where a dry period of intense evaporation generally occurs.
Finally, the last assumption may explain that kaolinite and albite could coexist in different size-fractions because weathering was still not able to destroy all albite. These rock substrates which are mainly made up of fine to very fine fractions, mainly silt and clay particles, are not supposed to be substantially affected by the grain size distribution of the sediment in agreement with Dinis & Oliveira (2016). Moreover, very fine grained fractions in the profiles may be depleted due to loss process controlled by diverse physical factors driven by topography (Tardy et al., 1973), remaining in the soils only the coarser fractions. This is directly linked to mechanical weathering and erosion rates. This last explanation associated to intense erosive activity requires great mineral similarity among weathered and fresh substrates, together with a low development in the soil profiles due to a continuous renovation-denudation in the recycling.
No less important is the narrow relationship which links geochemical composition with the contrasting climatic conditions. Dinis et al. (2020) and Perri (2020) have recently showed how chemical indices act as good proxies for chemical weathering to reconstruct past climate conditions. Dinis et al. (2016 and 2017) have also investigated the roles played by distinct processes on the weathering intensity and the palaeoclimatic conditions, demonstrating the composition of clay is strongly influenced by climatically driven weathering. Otherwise, Perri (2018) has shown higher abundance of kaolinite related to warm-humid conditions. Moreover, Perri (2020) released medium-low CIA values for similar climatic areas, in terms of mean annual temperature and precipitation, which underwent moderate-weak chemical weathering similar to this study. The results do not differ a lot from the values found in this study for the CIW, CIA and PIA; quite similar too to those obtained in previous works of Scarciglia et al. (2016) or Perri (2016).
In the light of the chemical indices and the linkages showing good relations with geochemical data, in this particular case, the albite hydrolytic alteration weathering by acid dissolution is still the main hypothesis supporting the presence of kaolinite in the most altered investigated soil profiles. Perri et al. (2015) demonstrated in granitoid weathering simulations the CO2-controlled dissolution of albite-rich plagioclase is the most important reaction, followed by the dissolution of K-feldspar, biotite, chlorite, and muscovite, in order of decreasing importance. In fact, differential chemical weathering occurring in the acidic solution of soils seems to better explain this way of alteration. The acidic solution from the oxidation of both, organic matter, quite important in the composition, and sulphides, abundant in the rocks, could explain the ways of alteration, supporting the presence of such abundant kaolinite. Some authors have already demonstrated the influence of organic matter in the acid dissolution to explain kaolinite presence (Gallardo Lancho et al.1976; Romero et al. 1992, Fischer et al., 2009; Li et al., 2014; Emberson et al., 2016; Ling et al., 2016). Solute transfer and elements mobility by acidic percolation of soil solutions should be studied further in order to clarify this assumption. So that, solute transfer and elements mobility by acidic percolation of soil solutions and leaching data should be studied further to give a concluding explanation like in other studies supported by Cronan (1985), Bain (2007) or Di Figlia, et al. (2007).
Conclusions
The identification of primary phyllosilicates and their coexistence with other secondary clays gives an enlighten clue indicative of possible different weathering processes affecting the soil profiles. Particularly, special emphasis is given to the albite occurrence, which is found coexisting with kaolinite in most of the mineral sequences. Kaolinite can be directly inherited in soils from parent rocks and partially due to mineral alteration, preferably from albite. In addition, since both could coexist in different size-fractions they are differently affected by weathering. So, it may be concluded that the geochemical composition must be also substantially affected by the grain size distribution of the sediment. Moreover, good correlations between chemical indices and element ratios, may indicate kaolinite in this case should come from the alteration of plagioclases during weathering.
The traditional chemical indices have also helped to understand different chemical weathering processes taking place. PIA, CIW and CIA have resulted an essential tool to infer some assessment due to the lack of other more specific data; giving a measure of plagioclase differential weathering; they together with, Al2O3/Na2O and K2O/Na2O, have also supported that kaolinite comes from the gradual alteration of albite mainly due to acid hydrolysis.
The differential weathering and albite alteration detected in these profiles is in agreement with the mobility of major elements leading to a general loss of bases (Na2O, CaO, K2O and MgO). In this work, the relations between the parent rocks composition and soils can easily assist in determining the bases loss and the K2O enrichment from the bottom to upper parts in the soil profiles. In general, a slight enrichment in silica and iron and aluminium sesquioxides attributed to the advancing process of weathering in agreement with most of findings show. However, Al2O3 in some profiles is slightly less abundant in soils than in parent rocks, probably because Al2O3 in the finest clay fractions is being lost by physical processes. Similarly occurring with SiO2, firstly enriched in the soil profiles when weathering advances and, later, leached out of the profile. Hence, besides chemical weathering, other physical or mechanical processes, due to erosion and removal, are expected to take placein such favorable topographic conditions. This together with a low development detected in the soil profiles leads to think of a continuous renovation-denudation in the recycling. In short, several factors, not only data limited to geological source areas and the critical lithology control, mainly based on geochemical and mineralogical composition, but also morphology and topography, climatic registers, or even vegetation interactions, may be assessed on weathering research.