Introduction
With the development of industry and manufacture, the amount of toxic and harmful substances in wastewater has gradually increased. The activated sludge method is the most widely used treatment technique for municipal and industrial sewage around the world. At present, more than 90% of the municipal wastewater is treated using the activated sludge method (Xiao et al., 2017; Yuan et al., 2019; Zheng et al., 2019). However, the main drawback of this approach is that it produces a huge amount of surplus sludge, which contains a lot of toxic substances and excess nutrients, including pathogenic microorganisms, parasite ova, viruses, synthetic organics, heavy metal ions, salts, nitrogen, phosphorous, potassium, etc. (Fei et al., 2019; Mazeed et al., 2020; Poot et al., 2016; Xia et al., 2019). The organic materials in the remaining sludge are easy to spoil and release a foul odor that pollutes the environment and causes discomfort to the senses. The pathogens in sludge, including various pathogenic bacteria, parasites, and viruses, can spread disease and pose a potential threat to human and animal health (Chakraborty et al., 2020; Zeng et al., 2019). Excess nutrients in the remaining sludge, such as nitrogen, phosphorus, and potassium can cause eutrophication and lead to algae blooms once they enter surface water bodies, which negatively impacts the environment (Fang et al., 2020; Hu and Gao, 2020; Zittel et al., 2020).
Nowadays, researchers are paying more attention to sludge reduction with modern techniques, such as physical, chemical, and biological methods, promoting the hydrolysis of sludge, which improves the efficiency of acid and methane production. However, sludge hydrolysis is a rate-limiting step in anaerobic digestion. Lysis-Recessive Growth (LRG) is a method for improving hydrolysis, which is easy to operate, simple to implement, and relatively mature. It involves breaking the cell membrane and solid cell wall of the microorganisms in the sludge, thus releasing organic matter into the liquid phase, increasing the organic load of sludge (F/M), and shortening the anaerobic digestion time of the sludge. LRG requires adding the pretreatment sludge to an anaerobic reactor for fermentation.
Heating excess sludge can effectively foster the dissolution of a large number of organic substances in microbial cells, making the microbe cells bigger and increasing the pore size of the cell membranes, which facilitates the transfer of a large amount of organic matter in microbial cells to the liquid phase for it to become soluble organic matter (Wu et al., 2020). When the alkali is added, the hydroxyl ion (OH-) plays a key role in destroying sludge flocs. In addition, it can also hydrolyze some proteins and nucleic acids, so that the original insoluble organic matter in the sludge turns into soluble substances transferred to the liquid phase. The addition of alkali can also reduce the resistance of the cell wall to temperature and accelerate the release of substances in the sludge (Feria-Díaz et al., 2016). Zheng et al. (2019) used a thermal-alkali pretreatment to treat straw and fermentation waste, and they found that a cumulative gas production above 80 °C can be increased by more than 40% when compared to a heat treatment only 80 °C, as well as by 72% in comparison with no-heat treatments (room temperature). The thermal-alkali method can effectively crack the sludge, which has some advantages in the process of anaerobic fermentation to produce acid. Furthermore, the addition of potassium ferrate can oxidize the surface film of the sludge micelle and destroy the structure of the film layer to release the material within it (Pabón and Gelvez, 2009). Furthermore, high temperatures could change the sludge structure, reduce the shear resistance capacity of flocculate, and make the organic suspended solids in water more soluble. Agitation has a positive effect on cell dissolution rate, as it enhances mixing between sludge and acid, alkali, and potassium ferrate.
In this study, the impact of temperature, pH, potassium ferrate dosage, reaction time, and stirring conditions on the dissolution of residual sludge was explored via the response surface methodology in order for the sludge cell dissolution rate to reach optimal conditions. With these optimal conditions, the model can efficiently guide the sludge reduction of pharmaceutical wastewater.
Materials and Methods
Experimental samples
The wastewater and sludge used in this study were taken from a solid-liquid separation tank in a pharmaceutical wastewater treatment plan in Shanghai, China, which mainly deals with high concentrations of pharmaceutical wastewater and has a daily processing capacity of 150 m3 in addition to a hydraulic retention time of 6 h. The sampled sludge was black with no serious bad odor. The composition of the sludge is shown in Table 1.
Experimental procedures and measurement methods
The experiment was conducted in a common laboratory. Samples from the pharmaceutical wastewater treatment plant were stored in plastic bottles and used for experiments either right away or after 5 h of being kept in a refrigerator at 4 °C. The wastewater sample was first evenly shaken, and about 300 mL of wastewater were poured into a 500 ml beaker. The pH value was adjusted using the 1:9 ratio of hydrochloric acid or 4 M of sodium hydroxide solution, and its value was obtained with a pH meter. Next, potassium ferrate was added into the wastewater sample, which was put in a blender for full mixing. The heating mode of the blender was also switched on in order to achieve the designated temperatures and start reactions. The 46 detailed test parameters are listed in Table 2. After the pretreatment, three wastewater samples of equal volume were put in a centrifuge for 30 min with a rotation rate of 1 500 rpm.
To measure the soluble chemical oxygen demand (SCOD), the supernatant was filtered through a 0,45 pm filter paper and diluted in a ratio of 1:20 using deionized water. The solution was then injected into a digestion tube filled with a solution of 6 ml of silver sulfate (10 g/L) and 1 ml of potassium dichromate. The digestion tube was mixed well and digested for 15 min in a microwave digestion instrument (Lanzhou Lianhua Environmental Protection Technology co., LTD, 5B-1B). The absorbance value of the solution was measured using an ultraviolet spectrophotometer (L6) and recorded to compute for supernatant COD values.
The total chemical oxygen demand (TCOD) was measured by following the SCOD procedure, albeit without filtration, and the diluted wastewater samples (1:20) were directly injected into the digestion tubes.
Three equal pretreated sludge samples were injected into centrifuge tubes and were centrifuged for 30 min at 1 500 rpm. The precipitate in the centrifugal tube was filtered using a suction filter machine (Bonsi Instrument Technology co. LTD), and dried to a constant weight in a crucible (Shaoxing Galaxy Machinery Instrument co. LTD, 101-0B) at 105 °C. The suspended solids (SS) value was finally obtained by weighing the dried samples on an analytic balance (Shanghai Yueping Science Instrument Co. LTD, FA2004B).
Data analysis
The equation for calculating the sludge dissolution rate (W) is expressed below:
where SCODt is the supernatant COD value after pretreatment, SCOD0 is the original supernatant COD value, and TCOD0 is the original TCOD value.
The data was analyzed by the response surface method of the Design-Expert V8.0.6.1 software. There were four main types of response surface designs, as well as a Box-Behnken design. A three-level, second-order response surface version of the Box-Behnken design was adopted in this research. The Box-Behnken design combined mathematical and statistical methods to determine the test design scheme according to its contents and purposes. The experiment was optimized by means of an analysis of variance, the estimation of the standard deviation and the signal ratio, and a regression analysis.
Results and discussion
Response surface optimization analysis
The synergistic effect of temperature and pH on the sludge dissolution rate is shown in Figures 1a and 1b for a reaction time of 6,5 h and a potassium ferrate dose of 5,5 mg/g SS with stirring. There were two regions (green) with a cell dissolution rate greater than 20%. In zone 1, the cell dissolution rate was more than 20% when the temperature ranged from 20 to 44 °C and the pH value was between 4 and 4,6. In zone 2, the cell dissolution rate was also greater than 20% when the temperature was between 32 and 80 °C and the pH value between 8 and 12. When the temperature ranged from 68 to 80 °C and the pH was between 11,5 and 12, the cell dissolution rate was above 40%. As shown in Figures 2a 2b, when the pH value was fixed at 8 and the potassium ferrate dose was 5,5 mg/g SS with stirring, the cell dissolution rate of the sludge did not significantly increase with temperature and time. The cell dissolution rate of the sludge was up to 18% when the temperature was between 50 and 68 °C and the reaction time was between 3,5 and 7,5 h. Therefore, the effect of the reaction time on the cell dissolution rate was not significant.
The synergistic effect of temperature and potassium ferrate dosage on the sludge dissolution rate is shown in Figures 3a and 3b for a pH value of 8 and a reaction time of 6,5 h with agitation. The sludge dissolution rate was more than 15% when the temperature was between 24 and 80 °C and the potassium ferrate dose ranged from 1 to 8 mg/g SS. The cell dissolution rate was above 20% when the temperature was between 37 and 80 °C, with a potassium ferrate dose between 1 and 4 mg/g SS. The results show that the rate of sludge dissolution did not always increase as the potassium ferrate did. As shown in Figures 4a and 4b, the dissolution rate of sludge was more than 20% when the pH value ranged from 9 to 12 and the reaction time was between 1 and 12 h. The cell dissolution rate was above 30% when the pH value was in the range of 11,5-12 and the reaction time was between 1 and 12 h. These results show that the effect of the reaction time on the cell dissolution rate was not significant in this case.
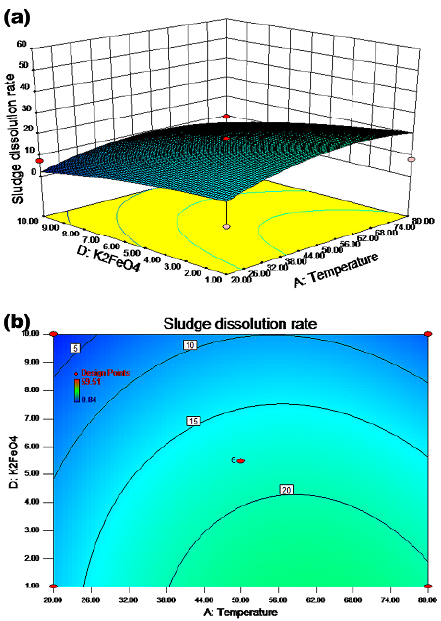
Source: Authors
Figure 3 Response surface (a) and contour (b) maps: potassium ferrate dosage and temperature
The synergistic effect of the pH value and the potassium ferrate dosage on the sludge dissolution rate is shown in Figures 5a and 5b for a temperature of 50 °C and a reaction time of 6,5 h with stirring. There were two regions with relatively high cell dissolution rate. In zone 1, the cell dissolution rate was more than 20% when the pH value changed from 4 to 8 and the potassium ferrate dose varied from 1 to 3,5 mg/g SS. In zone 2, the cell dissolution rate was greater than 20% when the pH value increased from 8 to 12 and the potassium ferrate dose increased from 1 to 10 mg/g SS. When the pH value was between 11,5 and 12 and the potassium ferrate dose was within the range of 1-10 mg/g SS, the cell dissolution rate was above 30%.
The synergistic effect of the reaction time and the stirring conditions on the sludge dissolution rate is shown in Figures 6a and 6b for a temperature of 50 °C, a pH of 8, and a potassium ferrate dose of 5,5 mg/g SS. According to the results, the cell dissolution rate improved; it increased by about 10% with stirring.
Fitting results and regression equation
Experimental data points were randomly scattered on both sides of the predicted straight line (Figure 7a), thus indicating an acceptable fit. The following quadratic multinomial regression equation for the sludge cell dissolution rate was obtained via Design-Expert for experimental design and multivariate analysis:
where P is the sludge dissolution rate, x1 is the reaction temperature, x2 is the solution pH value, x3 is the reaction time, x4 is the potassium ferrate dosage, and x5 is the agitation condition (0 for no stirring or 1 for stirring). An F-value of 3,16 with a p-value (Prob>F, less than 0,5) was obtained from the statistical test using the aforementioned model, which indicates a nonlinear relationship between the four factors and a significant difference among them. Said factors had a significant effect on the residual sludge dissolution rate, and there was some interaction between them. The R2 value regarding the fit of the regression model was 0,72, which was acceptable. The signal ratio was represented via Adeq Precision, which could be used for simulation purposes when it is greater than 4. In this study, Adeq Precision showed a value of 7,20, which was large enough to simulate the design.
Three repetitive experiments were conducted at 21,58 °C as well as with a pH = 4, a potassium ferrate dose of 1 mg/g SS, a reaction time of 4,07 h, and stirring, which are the optimal conditions given by the mathematical model. The obtained residual sludge cell dissolution rates were 52,78, 55,12, and 51,02%, which is listed in Table 3. The results showed a standard deviation of 2,06 and were very close to the 54,36% predicted by the software. Therefore, the reliability of this model was as expected, and the fitting diagram of the predicted values vs. the actual ones is shown in Figure 7b. According to Table 3, the real value under the best experimental conditions was very close to the simulation value obtained by the software, with a low error rate, thus indicating the reliability of the model.
Mechanism analysis
Changes in the residual sludge cell dissolution rate could be clearly observed, which are represented by color changes in Figures 1-6 (Capela et al., 2017). The strength of the interaction between two factors is represented by the change in the contour line. An ellipse indicates that two factors interacted significantly, whereas a circle means the opposite. All seven diagrams in this study had ellipse shapes, thus indicating significant interaction effects between factors.
The interaction effect of the temperature and pH values on the residual sludge cell dissolution rate is shown in Figures 1a and 1b. The results in Figures 4a and 5a show that the effect of the pH value on the residual sludge dissolution rate was significant. A positive linear relationship was observed, where an increased pH value caused an increase in the cell dissolution rate. Like the pH, increasing the temperature improved the dissolution rate.
As shown in Figures 1-6, two factors had a synergistic effect and could achieve the optimal treatment conditions, albeit within a specific range. There was an interaction between pH and temperature, and an optimal region of cell dissolution rate was observed in the 3D diagram. On the lower left corner of Figure 1b, the cell dissolution rate was relatively high (more than 20%). The correlation of SCOD with the low alkaline condition was relatively small, but there was a quite high correlation under high alkaline conditions. A possible explanation could be that only the flocculation structure of the sludge could be destroyed when the amount of alkaline was low, unlike the cellular structure of the microorganisms. However, both structures could be destroyed simultaneously under high alkalinity to produce organic materials such as hydrolyzed proteins and carbohydrates. Originally insoluble organic matter in the sludge were also released from microbial cells and became soluble substances, so the SCOD concentration of the sludge increased (Wilson and Novak, 2009).
A large amount of dissolved total sugars, soluble proteins, and other organic substances were released when cells lysed after the residual sludge was heated. Some highly hydrophilic substances such as proteins, carbohydrates, and nucleic acids became other, likely smaller compounds during heating. For instance, the proteins prevalent in EPS can lose their normal shapes and even precipitate. Furthermore, high temperatures could change the sludge structure, reducing the shear resistance capacity of the flocculate and allowing the organic suspended solids to be more soluble in water.
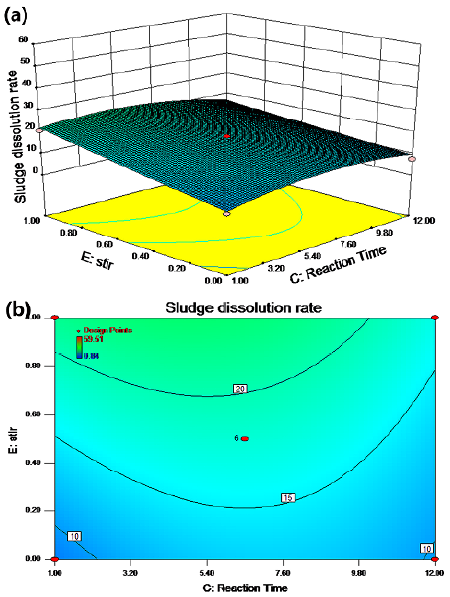
Source: Authors
Figure 6 Response surface (a) and contour (b) maps: agitation conditions and reaction time
Figures 2a and 2b show the effect of the interaction between temperature and reaction time on the residual sludge cell dissolution rate. A linear relationship with a relatively small slope between the cell dissolution rate and the reaction time was observed in the contour map. This indicated the insignificance of time, which was regarded as a secondary factor. A possible reason could be the dynamic equilibrium between the growth and decay of microorganisms over a relatively long period of time (Wang et al., 2013). A weak interaction between temperature and reaction time can be observed in Figures 3 and 4, and an elliptic region indicates a cell dissolution rate of more than 18%, when the temperature was between 50 and 68 °C and the reaction time between 3,5 and 7,2 h.
The results indicate that the addition of a moderate amount of potassium ferrate could improve the cell dissolution rate of sludge according to Figures 3a and 5a. However, based on the experimental observations, further addition of potassium ferrate (> 4 mg/g SS) does not increase the SCOD values. There were two possible mechanisms: (1) potassium ferrate oxidized the extracellular polymers of the sludge; or (2) potassium ferrate oxidized the cell membrane and reacted with some of the dissolved materials. When the oxidation and reaction processes were completed, the remaining potassium ferrate had nothing to react with. This explains why an excess dosage had no effect. Therefore, the optimal dosage of potassium ferrate was 4 mg/g SS when combined with other factors, such as temperature, pH, and reaction time.
The effect of the interaction between the reaction time and the pH value on residual sludge cell dissolution rate is shown in Figure 2b. The results showed that the cell dissolution rate of the residual sludge increased with an increased pH value. The cell dissolution rate was above 20%, as shown in Figure 5a, when the pH value was relatively low and the amount of potassium ferrate additive was lower than 4,5 mg/g SS. The cell dissolution rate was not affected by the amount of potassium ferrate and the reaction time when the pH value was over 10. The rate was more than 30% according to Figures 2b and 5a. The influence of the reaction time on the excess sludge cell dissolution rate was not significant, which was similar to the results shown in Figures 1b and 6a. From the contour map and 3D diagram, the effect of the interaction between pH and reaction time was not significant -based on the numbers and color change. Therefore, the pH value played a vital role in this reaction process.
pH adjustment is one of the key procedures during the sludge pretreatment process because it can break flocculate and cells in the sludge within a very short period. When hydroxyl was added (the pH value was increased), lipids, carbohydrates, and proteins were degraded into small molecules, such as fatty acids, polysaccharides, and amino acids, thus reducing the solid parts of sludge. EPS could be dissolved under high alkalinity, and the process not only involved chemical degradation, but also the ionization of OH-, which caused EPS to swell and dissolve greatly (Zhang et al., 2021). The microbial cell wall was destroyed when EPS was dissolved by strong alkalis, and a saponification process continued to react between the alkali and the lipids of the cell membrane. After the cell membrane dissolved, the intracellular materials and water were released from the cells. These could be utilized by other microbes. As a result, a higher pH value had an obvious effect on the cell dissolution rate, and the biochemical properties of the sludge improved (Chen et al., 2013; Hong and Li, 2011; Kelessidis and Stasinakis, 2012).
Although there are few studies that explore the reduction of sludge via response surface models, especially with regard to pharmaceutical wastewater, this model involved five factors, which was much more than that of the solar photo-Fenton process, which has an error value of 6,91(Kavitha et al., 2015). The mathematical model is helpful to find the optimal operation control conditions for the sludge reduction process. In addition, the error between the theoretical simulation value and the actual one was less than 6,34% with few dosages of potassium ferrate (1 mg/g SS), which meets the requirements of engineering applications. Therefore, this mathematical model can provide a processing method to reduce other kinds of sludge.
Conclusions
The sludge from a pharmaceutical wastewater treatment plant was treated in this study. The effects of temperature, pH adjustment, addition of potassium ferrate, time control, and stirring on sludge reduction were studied. By using the response surface method, a mathematical model was established. Based on the experimental data, the optimum experimental conditions were obtained by means of the mathematical model. At a temperature of 21,58 °C, as well as with a pH value of 4, a potassium ferrate dose of 1 mg/g SS, and a reaction time of 4,07 h with stirring, the cell dissolution rate of sludge reached 54,36% as predicted by the mathematical model. The triplicate cell dissolution rates of the residual sludge were 52,78, 55,12, and 51,02%. Thus, a high cell dissolution rate and a good sludge reduction effect were achieved in this study, and a relatively reliable mathematical model was established.