1. Introduction
Climate change is a natural process that has been accelerated by human influence due to the large amount of greenhouse gas emissions into the atmosphere. It is related to human development, population growth, and consumption patterns; this phenomenon, whether natural or anthropogenic, can generate serious consequences for the environment and humanity. Therefore, to prevent and mitigate this phenomenon’s negative impacts on the environment, including its effects on the hydrological cycle in a particular region, it is necessary to develop techniques with sufficient technical and scientific quality[1].
Water shortage conditions tend to impact the hydrological services offered by the Andean basins in South America, severely impacting the different sectors of existing populations, making it necessary for decision-makers to create innovative solutions at the basin level. Integrated water resource management (IWRM) is a concept that aims to promote adequate planning and management of the resource to ensure its sustainability and conservation[2] so that decision-making measures and tools can be established reducing water availability risks for current and future scenarios. It is important to highlight that over the years, as the risk of water scarcity increases, the demand for water solutions increases; approximately 55% of the world population will face a water resource crisis by 2050[3].
This crisis is related to aspects such as high levels of industrialization, population growth, and climate change; therefore, it is essential to take action to conserve water resources[2]. In Colombia, there is a constantly growing trend in agricultural areas. The demand for this sector depends on the climate (climate variability and climate change), which directly impacts the variation in demand and the water deficit of the sector[4]. The quality of life of the populations dependent on the agricultural sector would decrease dramatically in a water crisis, and negatively impact food security. In addition, there would be changes in the economic and productive sectors, with increases in the prices of products and a significant deterioration in crop yield[5].
Agriculture is one of the most vulnerable sectors to change in the future climate[6] and, as such, has been the subject of multiple studies in recent years[7-10]. Several quantitative assessments of vulnerability to climate change have been made through different analyses and comparisons of agricultural vulnerability in different regions of the world, generating composite indices based on sets of indicators[11]. Such indicators generally cover multiple dimensions of the concept of vulnerability, capturing the exposure, sensitivity, and adaptability of a system[12, 13].
In the case of Colombia, according to the Ministry of Agriculture and Rural Development, agricultural production at the national level has been affected by the occurrence of extreme weather events associated with climate change. The above has generated impacts of great magnitude at the regional level, due to changes in the rainfall regime and the behavior of temperature, causing floods, landslides on cultivated land, proliferation of pests, changes in cultivation cycles and greater seasonality in the productive territory[14]. In this investigation, the risk of a water supply failure in the agricultural sector was evaluated using hydrological modeling tools, to support decision-making about the satisfaction of consumptive demands in times of shortage, taking into account the influence of climate change scenarios[15].
1.1 Case study
The Balsillas river basin is located in the Cundinamarca department in Colombia, belonging to the Bogotá river basin, as presented in Figure 1. The Balsillas river is mainly formed by the currents of the Bojacá and Subachoque rivers, which are its main tributaries[16].
The basin has a total area of 624 km2, and the main channel is 68.1 km long. It begins at an elevation greater than 3,700 m.a.s.l. and ends over 2,500 m.a.s.l.; the average elevation of the basin is 2,730 m.a.s.l.
About the topography, there are mountainous reliefs in the northern and eastern parts that cover 42.5% of the total area, where heavily wavy to heavily broken and steep reliefs predominate; while in the central and southern regions of the basin, floodplains are located, covering 43.2% of the total area. The remaining 14.2% of the basin are areas with wavy to sloping relief[16]. The landscape in this area is flat with cold and mostly dry weather conditions. The main vegetation cover present in the area is primary forests, stubble, thickets, and paramo vegetation. As for the type of soil, those with fine textures susceptible to water erosion predominate when there is no vegetation. In addition, there are sedimentation and wetland filling processes that generate flooding risk due to overflow and flooding when water levels increase significantly[16].
The soils are rich in nutrients, allowing agro-industrial activities with pea crops, cape gooseberries, strawberries, tomatoes, and pastures. These activities are essential for employment generation and economic growth for the community[5].
The primary use of water in the basin is for economic activities related to agriculture, generating an approximate demand of 3.3 m3/s, which has caused a decrease in the water resource amount. Other important demands are the ecological flow, with a requirement of 0.15 m3/s, domestic consumption, with 0.062 m3/s, and finally, the lowest demand is industrial, which requires 0.024 m3/s of Balsillas river flow[16].
2. Materials and methods
The failure probability evaluation was developed by analyzing water resources management for different future scenarios. For this, future water availability was obtained through the water balance method using the Hydro-IDB tool, which is an integrated and quantitative system to simulate hydrology and water resource management in the Latin American and Caribbean regions, whose system applies the standardized Generalized Channel Load Factor (GWLF) model in conjunction with a new time delay-routing methodology (lag-routing)[17]. This tool integrates an analytical hydrography database (AHD) that contains information on the topology of the basins, stream stretches, land use and cover, soil types, precipitation, temperature, and observed stream flows, which represents an advantage for its application in regions with little information.
The hydrological model requires input data, such as precipitation and temperature. The calibration is done manually by trial and error, by changing the values of the curve number parameters (NC), the available water content in the soil (AWC), coefficient of recession (R), permeability coefficient (see page), ET factor of the growing season, ET factor for the latency season, and percentage of permeable cover. The general volume error, Pearson's correlation coefficient, modified Pearson's correlation coefficient, and Nash-Sutcliffe efficiency were used as efficiency indicators to evaluate the model’s efficiency.
As a future large-scale climate dataset, the CORDEX experiment database was used for different scenarios of greenhouse gas concentrations (CPRs), applying different performance metrics for the selection of the best climate model that would adjust to the weather conditions of the study area. Likewise, a statistical downscaling method based on chaos theory was also applied, which relates the future climatology of the selected large-scale climate model to the local data at basin-scale obtained through climatological stations.
The Chaotic Statistical Downscaling model (CSD), presented in[18] and evaluated in[1], is a new statistical downscaling method based on phase space reconstruction for different time steps, identification of deterministic chaos, and a general synchronization predictive model. We assessed the presence of deterministic chaos and the identification of the dynamic system movement type for different accumulation intervals by calculating the Lyapunov exponent for both the large-scale model and the local-observed system.
The CSD model has been used with relative success to predict both precipitation and temperature in different basins of Colombia, such as the Bogotá river basin and the Totaré river basin. As presented in[1, 18], the method was also compared with other downscaling techniques under three different error measures in these basins.
The process continues by next using AQUATOOL Decision Support System (DSS)[19], specifically, the module for simulating water resource systems management (SIMGES)[20] and the module for risk management evaluation (SIMRISK). Simulation in SIMGES consists of a conservative flow network optimized monthly by linear programming using the Out-of-Kilter algorithm[21] to maximize an objective function (demand satisfaction and storage of deposits) subject to mass conservation restrictions, the physical limits of flow transport in channels, and reservoir capacities. This simulation process for several scenarios is performed by running the SIMRISK model, which evaluates the short-, medium-, and long-term management risks for a given river basin[22]. The model will generate scenarios of multiple and future natural flows and simulate the management of the system according to the criteria previously defined for each scenario. This modeling is based on Monte Carlo simulation. This model’s results are probabilistic information that allows analyzing the number and severity of failures in the system[23].
Although there is a wide variety of models that solve problems related to water management and planning through simulation and optimization models, SIMGES and SIMRIKS were selected because they are user-friendly. Their use does not present excessive computational costs[24].
This methodology is presented in Figure 2, and it consists of the following steps once the series of future flows is obtained through hydrological modeling incorporating the influence of climate change for the study area:
With the series of flows generated, the water exploitation system’s current characteristics, and the system management rules, a simulation of future water resource management in the basin is carried out.
The results of the simulation are statistically analyzed to obtain the failure probabilities for the agricultural sector demands.
The information provided in the previous step determines the state of the system and supports the decision-making process on the failure probability’s admissibility.
When the probability is not accepted, new management alternatives are established that feedback to the management simulation (Step 2). The following steps are repeated until a new decision is made about whether the ruling is acceptable.
3. Results and discussions
3.1 Hydro-BID calibration and validation
As a result of the calibration, the parameters’ final values at the outlet of the basin were obtained. The following performance metrics were calculated daily and monthly: general error volume, correlation coefficient R, modified correlation coefficient, and coefficient of Nash-Sutcliffe efficiency.
From these results, it is evident that the calibration process was satisfactory, as shown in Figure 3. The performance metrics shown in Table 2 proved to be acceptable. Monthly values were found to be the best to determine that the model is functional, as these are within the ranges established by the Inter-American Development Bank (IDB).
It is important to note that changes in the calibration parameters significantly impact the simulated flows; therefore, as the curve number decreases, the flow rates decrease because the infiltration increases. Therefore, there is less runoff. Likewise, the water content in the soil (AWC) and the seepage influence the runoff’s magnitude because if they increase, there is a greater impact on the low flow rates, so the infiltration increases. With respect to the recession coefficient (R) when modifying it, the flow curve slope increases, so that the runoff is increased and, therefore the flow rates have higher values.
Regarding the validation, once the calibration has been carried out, the model is run again for a later period, between 01/01/2005 and 12/31/2009, and the results obtained showed a similarity in the behavior of the flow duration curve between simulated and observed data, as shown in Figure 4; however, as in the calibration, the modeled flows were overestimated.
Concerning the validation performance metrics in Table 3, the monthly values are more accurate than the daily data. They are within the ranges stipulated by the IDB, indicating that the model is functional.
The most sensitive parameters in the model are the curve number and the AWC since they are the ones that mainly control the amount of runoff generated throughout the basin. This is mainly because the curve number represents the level of waterproofing in the study area, due to natural or anthropogenic conditions, favoring, or not, the infiltration processes by precipitation received in the soil, and the AWC determines the volume of water that can be absorbed before the soil reaches its saturation point; all the water received in the area will become runoff from that moment.
3.2 Global Circulation Model (GCM)
The selection of the climate model that best fits the local conditions is made by comparing the error rates of the mean, maximum, minimum, standard deviation, correlation coefficient, wet/cold days, and warm/dry days for each model and CORDEX scenario. For precipitation, the climate model that best fit was the CORDEX experiment with the MPI-ESM-MR model. The values resulting from the comparison can be found in Table 4.
This model was chosen because there is a similar behavior in the interannual variation in multiannual monthly precipitation graphs (see Figure 5]. It also presents the best correlation of all evaluated models. In relation to the criteria of dry and wet days, the percentage of error is minimal, indicating a similarity between the data.
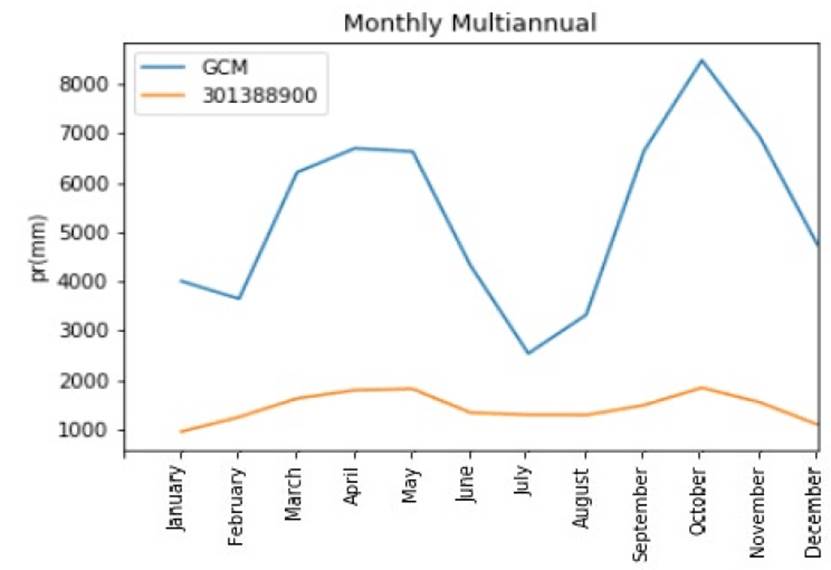
Source: Authors.
Figure 5 Comparison of GCM data vs. historical precipitation data for Sub-basin 301388900
For temperature, the climate model that best fits was the GCM experiment with the MPI-ESM-MR model[25]. The values resulting from the comparison can be found in Table 5. At the graphic level, an adjustment of the model data is observed with the data of the series observed in the basin [Figure 5, Figure 6].
3.3 Downscaling Results
In the case of precipitation, an increase is shown with respect to the average value for mid-century, around 2050-2075 (MOC), and by the end of it, 2075-2100 (EOC). In the case of RCP 2.6, an average increase of 1.83% is presented for the MOC and 4% for the EOC. For RCP 4.5, an increase of 6.53% is shown for MOC and 6.9% for EOC. Finally, for the RCP 8.5 scenario, there are 7.43% increases for MOC and 7.97% for EOC.
In relation to the temperature for the RCP 2.6 and RCP 4.5 scenarios, there are increases both for the mid-century (MOC) and for the end of the century (EOC), on average 0.17% and 0.23%, respectively. Compared to RCP 8.5 the temperature shows a decrease of -0.43% for the MOC and -0.60% for the EOC.
By applying the climatological data obtained through the scale reduction method and then integrating them into the HydroBID model, the flows were obtained for the periods from 2014 to 2040, 2040 to 2070, and 2070 to 2100. When carrying out this modeling, different variations were obtained that directly affect the basin’s hydrological regime. As can be seen in Figure 7, the flows gradually increase for each RCP over the period between 2014 and 2100. In the case of RCP 8.5, the largest flows are present since the registered peaks reach approximately 7 m3/s, so it is inferred that the runoff processes are higher. However, the flows for RCP 4.5 fluctuate between 1 at 3 m3/s, and there are certain peaks close to 5 m3/s towards the period from 2030 to 2034. For RCP 2.6, the basin’s flow levels have the smallest values where the most significant minimum flows are presented; the results reach approximately 2 m3/s [Figure 7].
As presented in Table 6 for RCP 2.6, the average of the flow data is close to 1.28 m3/s, which corresponds to an increase of 5.2% compared to current conditions. However, in reference to RCP 4.5% of the simulated flows correspond to 1.24 m3/s, which represents an increase of 2.1%. Finally, for the scenario corresponding to RCP 8.5, the flow data presents an average of 1.29 m3/s, indicating an increase of approximately 6% compared to the basin’s historical flow data.
3.4 Modeling in SIMGES and SIMRISK
Figure 8 shows the topology of the Balsillas river basin in terms of demand points for agricultural use identified in the area. The structure begins with the main contribution in the upper part of the basin, followed by identifying 20 points of consumption along the river sections. The results for modeling in SIMGES are presented in the graphic and through tables in which the deficit reached by each demand point can be seen. 20 results were obtained by identifying that under the established assumptions of flow and demand for the study, deficits were presented in 9 of all consumption points. The average deficit value is 0.0004 Hm3, which corresponds to 30% of the total demand at each point. Table 7 shows the maximum deficit reached in each of the climate change scenarios.
The probability of failure to guarantee the total demand is below 40% on average. Considering the 20 consumption points, as shown in Figure 9, the percentages of failure probability range from 20 to 40% in climate change scenario, indicating that the highest percentage of failure occurs in November and December and the lowest between April and May. It should be noted that the points with a higher probability of failure are present in the middle part of the basin. However, some points do not present any probability of supply failure; in addition, these failures are generally presented on a 30-60% supply.
4. Conclusions
For the Balsillas river basin, agricultural production changes are estimated due to variations in temperature and precipitation, which directly impact crop yields. Under the conditions of scenarios RCP 2.6, RCP 4.5 RCP 8.5, the climatic variables will increase between the years 2035 to 2065, so that these conditions are expected to influence the production of representative crops in the area.
The climate change conditions of each RCP scenario influence the availability of the basin to supply water to the different demand points. For the years between 2035 and 2065, an increase in the water supply is estimated. For RCP 2.6, the flow data is expected to present a 5.2% growth, corresponding to an average of 1.28 m3/s. For the RCP 4.5, a 2.1% rise is expected, which corresponds to an average of 1.24 m3/s, and finally, in RCP 8.5, the flow data presents an average of 1.29 m3/s, indicating a build-up of approximately 6%. However, it also predicts an increase in agricultural use consumption due to the increase in the Gross Domestic Product (GDP) for future periods, so the supply will not be sufficient for the projected demand.
The deficit corresponds to about 30% of the total demand at each point, which corresponds to 0.0004 Hm3, so it is estimated that for 2035-2065 the basin will not fully meet the demands for agricultural use in the area, since there is a risk of failure at certain points; however, the probability of this happening is below 40%. Only 9 of the 20 points of agricultural demand presents a risk of failure.
The presented methodology provides the possibility of generating an adequate analysis of the water supply and demand relationship, which is essential for water planning and management in water deficit basins since it allows inferring rules and policies for the use of the resource. In addition, the introduction of water resources management rules that incorporate the risk associated with the decision and provides decision-makers with the security and confidence to contemplate the system globally or each of its components.