Introduction
Papaya (Carica papaya L.) is a popular and economically important fruit species in tropical and subtropical countries (Valencia et al., 2017). It is consumed fresh and processed and has medicinal properties worldwide, ranking fourth among the most produced fruits (Özkan et al., 2011; Teixeira et al., 2007; Valencia et al., 2017). Mexico has the soil and climatic conditions for developing this crop (SAGARPA, 2017). The United Nations Food and Agriculture Organization (FAO) indicated in 2018 that Mexico ranks fourth in production with 720,033.26 t and first among exporting countries with 168,000 t in the same year.
Papaya is produced on large outdoor surfaces. It is estimated that in 2017 there were 449,000 hectares planted worldwide, with 19,000 hectares in Mexico (SAGARPA, 2017). When papaya production is carried out outdoors, pest and disease control is complicated, representing a high cost to the production system. So, plantation in greenhouses is proposed as a viable alternative to achieve better management of biotic and abiotic factors, in addition to longer production cycles (Honoré et al., 2019). Crop establishment under greenhouse conditions has been conducted experimentally (Farina et al., 2020; Özcan et al., 2011), and it is incipient commercially; only some countries have done so. One of the drawbacks is the height reached by the plants, so it is necessary to implement a tall enough structure not to interfere with the development of the plant.
The production of papaya in greenhouses has been carried out commercially in the European Union, working with an agronomic model for its large-scale production. The results are promising since population densities from 2,000 to 2,500 plants per hectare are obtained, with yields of 10 to 12 kg/m2, where fruit quality and disease control are significantly controlled (García, 2016).
Tolerance to salinity depends on each species. In the case of papaya, it has been reported to be moderately sensitive (Grieve et al., 2012; Villafañe, 2000), while Elder et al. (2000) indicated that it is very sensitive. More than a matter of species, it depends on the genotype. Subhas et al. (2007) found a diversity in values from 0.6 to 4 dS/m in assessing the tolerance to saline stress of six papaya genotypes.
Among the processes that salinity affects are photosynthesis, enzymatic synthesis, stomatal conductance, reduction of plant height, number of leaves, and foliar area (Orosco et al., 2018) by inhibiting cell division and expansion (Parés & Basso, 2013; Parés et al., 2008).
As another response to stress, the plant raises the chlorophyll content according to the increase in saline stress, which occurs thanks to the increase of nitrogen compounds. In the same way, it generates physiological defense mechanisms, inducing an osmotic and hydric adjustment to avoid ionic toxicity; due to all the above, osmotically active organic compounds such as proline, glycine-betaine, carbohydrates, proteins, among others, are synthetized (Becerra et al., 2019; Jamil et al., 2018). At the nutriment level, some elements such as magnesium and calcium do not suffer any imbalance in the plant. However, others, such as phosphorus and potassium, tend to decrease, sometimes reaching critical levels in the plant; on the contrary, nitrogen content tends to rise as a response to salinity tolerance (International Plant Nutrition Institute [IPNI], 2009. Therefore, this research aims to identify nutrient solutions that reduce plant height and evaluate the salinity effect on chlorophyll concentration, proline production, and foliar nutrient concentration.
Materials and methods
The study was conducted in a semi-tunnel greenhouse, with a passive zenithal and lateral ventilation, between March and November 2019. It is located in the Academic Unit of Agriculture of the Autonomous University of Nayarit, at km 9.0 of the Tepic-Compostela highway, in the municipality of Xalisco, State of Nayarit, Mexico, with coordinates 21°25′ North Latitude and 104°53′ West Longitude.
Plant material
Maradol papaya seeds were used and sown in 200-cavity trays. Transplantation was performed when they had two leaves (20 days after emergence) in pots of 20 L capacity using tezontle as a substrate, with a particle less than 2.0 cm in diameter.
Irrigation
A drip irrigation system was used, with a daily expenditure of 300 mL of solution during the first month. It was subsequently raised to 400 mL during the second month, 1.0 L from the third month, 2.0 L in the fourth month, and 4.0 L from the fifth month until the end of the study. To avoid the accumulation of salts, only water was weekly applied until the draining EC resulted in 0.5 dS/m. Fertigation-grade soluble fertilizers, potassium nitrate, calcium nitrate, potassium sulfate, mono potassium phosphate, magnesium sulfate, and a mixture of micronutrients (Micromix® Mexico) were used.
Treatments
The treatments were different concentrations of the universal nutritive solution proposed by Steiner (1961): 50, 100, 150, and 200 % (Table 1). The application of the treatments began at the time of transplantation.
Experimental design
The experimental design was completely randomized with four treatments and ten repetitions, obtaining a total of forty experimental units.
Evaluated variables
Plant height
Height was recorded using a tape measure (cm). The measurement was made at 87, 94, 101, 108, 172, and 236 days after transplantation (DAT), considering the stem start to the apical meristem.
Number of leaves
The number of mature leaves per plant was counted at 87, 94, 101, 108, 172, and 236 DAT.
Stem diameter
The stem diameter was measured at the height of 5.0 cm from the base with a Truper® digital vernier; the result was expressed in mm. The measurement was made at 87, 94, 101, 108, 172, and 236 DAT.
Leaf area
A fully developed leaf was chosen from each plant and evaluated using the portable leaf area meter (CI-202). This variable was recorded at 94, 108, 140, 172, and 236 DAT.
Chlorophyll a and b concentrations
At 236 days after sowing, ten fully developed leaves were selected by treatment, dried, lyophilized, and pulverized to determine the concentration of chlorophyll a and b, using the methodology established by Hiscox and Israelstam (1978): chlorophyll a = (12.7 x abs 663 nm-2.7 x abs 645 nm) and chlorophyll b = (22.9 x abs 645 nm-4.7 x abs 663 nm). With the spectrophotometer (Jenway, 6320D), the absorbance was measured at wavelengths of 645 and 663 nm, and acetone 99.5% (JT Baker) samples were used at 80% as a reference.
Proline content
Proline quantification was performed according to Bates et al. (1973). A sample of the leaves processed for determining chlorophyll was used.
Foliar nutrient concentration
The concentration of nitrogen, phosphorus, potassium, calcium, and magnesium was determined in fully developed leaves at 236 DAT. The corresponding samples were washed and dried on a stove with forced air circulation at 70 ºC up to constant weight, ground with a stainless-steel mill (IKA® A11, Germany), and weighed on an analytical balance (Ohaus of 0.0001 g precision). Subsequently, the relevant chemical analyses were performed. Total nitrogen was determined by acid digestion through the Kjeldahl method in its micro version (< 100 mg). The rest of the macronutrients were extracted by wet digestion, with a mixture in a 2:1 ratio of H2SO4 and HClO4 (Alcántar-González & Sandoval-Villa, 1999). Its quantification was performed with a Varian® atomic absorption spectrophotometer (Palo Alto, California, USA) for K, Ca, and Mg, while the concentration of P was determined by the colorimetric method with a Hach DR 2800 spectrophotometer® (Ames, Iowa, USA).
Results and discussion
Plant height
The effect of the treatments was observed from day 101 after transplantation. At the end of the experiment, the salinity (4 dS/m) reduced the plant height by 27% compared to other treatments with a statistically similar behavior (Table 2).
A similar study conducted by Parés and Basso (2013), who evaluated the salinity effect on Maradol papaya plants, reported that the significant effect of the EC began when the value was greater than 2.0 dS/m. This study differs from this research in the elements used for salinity induction since NaCl was employed. In contrast, in this study, macronutrient elements were used in ion balance, which may explain why the salinity concentration generated by Steiner nutrient solution in EC from 1 to 3 dS/m has not affected the plant’s physiological processes compared to the salinity generated by NaCl. The adverse effect caused by salinity stress depends on the time the plant is subjected to such conditions since the longer the time, the more perspiration occurs, and the tissues tend to accumulate more salts (Negrao et al., 2017), reaching clearly visible phytotoxicity. Another factor influencing is the level of salinity applied to the rhizospheric medium and the types of ions that cause salinity. In this sense, the Maradol papaya can moderately tolerate salinity, as indicated by Campostrini and Glenn (2007).
Table 2. Salinity effect on the height of Maradol papaya plants, established in greenhouse and hydroponics
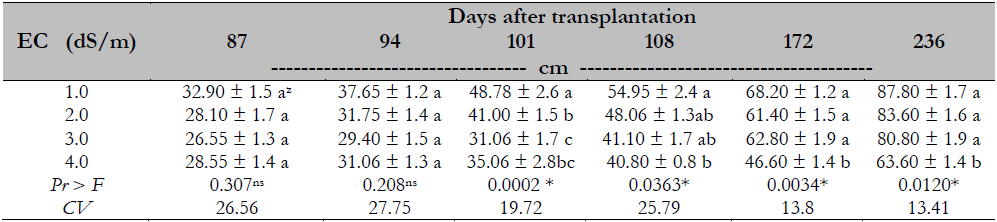
Note.CV = Coefficient of Variation; zvalues with identical letters in the same column indicate that they are statistically equal groups (Duncan’s α ≤ 0.05); ns = not significant; * = significant difference.
Source: Elaborated by the authors
Number of leaves
The salinity effect on the number of plant leaves shows that the higher the EC, the smaller the number of leaves in the papaya plant. This event is derived from the stress caused by salinity, as observed in the range evaluated from 1 to 4 dS/m (Table 3).
Table 3. Salinity effect on the number of leaves of Maradol papaya plants
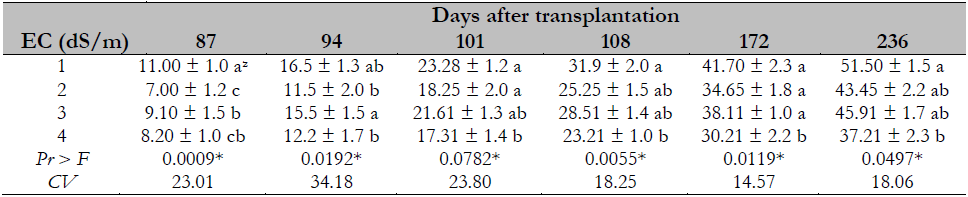
Note.CV = Coefficient of Variation; zvalues with identical letters in the same column indicate that they are statistically equal groups (Duncan’s α ≤ 0.05); ns = not significant; * = significant difference.
Source: Elaborated by the authors
The results obtained coincide with other studies indicating a significant decrease in the number of leaves due to |the salinity effect, such as Muñoz-Ramos et al. (2004), where salinity decreased the number of leaves to 16% of in red pepper (Capsicum annuum L.), Francois (1996), who found a reduction of 20% in sunflower (Helianthus annuus L.), and Dolatabadian et al. (2011), who observed a reduction of 25% in soy (Glycine max L.). The above results from salt stress decreasing stomatal density (Parés et al., 2008) and therefore affects photosynthesis, decreasing these plants’ growth and development.
Stem diameter
The analysis of variance and testing of Duncan’s means (P ≤ 0.05) indicated a difference and an effect of treatments on stem diameter. The stem diameter was reduced when the EC was greater than 2.0 dS/m from 101 days after transplantation (Table 4).
Table 4. Salinity effect on stem diameter of Maradol papaya plants
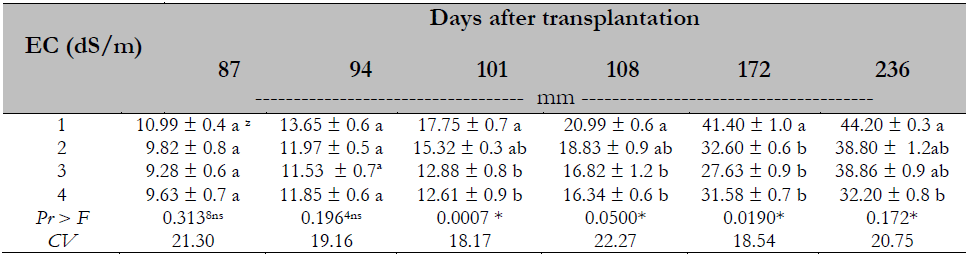
Note.CV = Coefficient of Variation; zvalues with identical letters in the same column indicate that they are statistically equal groups (Duncan’s α ≤ 0.05); ns = not significant; * = significant difference.
Source: Elaborated by the authors
The results in this research coincide with those obtained by Parés and Basso (2013) in Maradol papaya, Muñoz et al. (2004) in red pepper (Capsicum annuum L.), and Mahmood et al. (2009) in Acacia ampliceps Maslin, where stem diameter was reduced up to 51%. This effect is related to the decrease in stomatal density and stomatal closure mentioned by Parés et al. (2008). In this research, the stem diameter was finally reduced by 27 % compared to the diameter recorded by plants submitted to 1.0 dS/m. Because of the herbaceous consistency of the papaya stem, the plants are affected by the winds. As they must withstand the great weight of fruits, stems break or burn and fruits fall or reach contact with the ground when hurricane winds occur, reducing yield (Arrieta & Carrillo, 2002). The preceding can be seen as a disadvantage, but it would not be so if this technique were applied under protected farming conditions.
Leaf area
In the variable leaf area, it was observed that the higher the EC, the smaller the leaf area; this effect is observed at all sampling times. The leaf area decreased when the EC was higher than or equal to 2.0 dS/m, and the differences are most evident towards the end of the experiment, where as the EC increased, leaf area decreased significantly; there was a 54% decrease in the area at a EC of 4.0 dS/m compared to 1.0 dS/m (Table 5).
Table 5. Salinity effect on the foliar area in Maradol papaya plants, established under greenhouse conditions and hydroponics
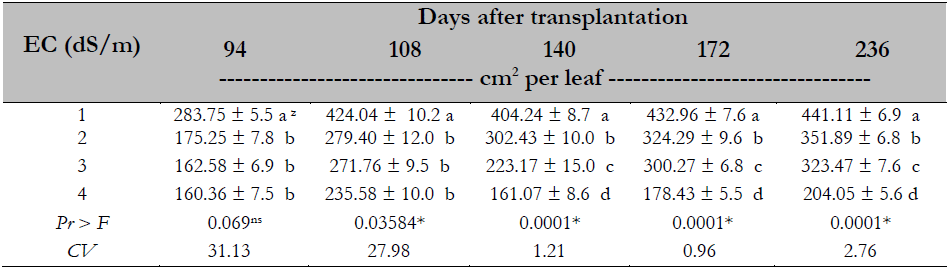
Note.CV = Coefficient of Variation; zvalues with identical letters in the same column indicate that they are statistically equal groups (Duncan’s α ≤ 0.05); ns = not significant; * = significant difference.
Source: Elaborated by the authors
The decrease in leaf area concerning the increased salinity in the treatments studied is consistent with studies conducted by Parés et al. (2008), showing that at EC higher than 2.0 dS/m, leaf area decreases to 36% in the papaya plant. This same effect was reported by Veli and Halit (2009) in melon with a 44% reduction of leaf area caused by the EC of 8.0 dS/m. The above is a plant strategy to counteract the adverse effects produced by salinity. Reducing leaf area decreases water loss by transpiration, suppressing the negative effect caused by this stress.
Similarly, research on tomatoes indicates a decreasing response in leaf area proportional to the plant salinity increase since the reduction in water absorption capacity caused by osmotic stress causes a loss in the foliar expansion (Ruiz et al., 2012).
Chlorophyll a and b concentrations
The salinity effect on the papaya plant shows that the higher the EC, the higher the chlorophyll a and b concentration, as observed in the range evaluated from 1 to 4 dS/m (Figure 1).
Studies by Ding et al. (2018) demonstrated that chlorophyll a and b content reported an increment of 100% when EC changed from 0.3 to 9.6 dS/m, in addition to a lower content of chlorophyll b, generated to improve tolerance to salinity, thus contributing to changes in photosynthesis. This photosynthetic process is initiated by the absorption of light by photosynthetic pigments (chlorophyll a, b, and carotenoids) of the antenna complexes (Conceicao et al., 2017), which is also consistent with the results obtained.
Proline content
The proline content in the papaya plant increases significantly as the salinity increases, as observed in the evaluated range from 1 to 4 dS/m. The proline concentration is strongly proportionally related to the EC (r = 0.99) (Figure 2).
The results of this research are consistent with Jamil et al.’s (2018), who found an increase of proline from 3 to 55 mg/g when EC changed from 1.0 to 4.0 dS/m in red pepper (Capsicum annuum L.), and Becerra et al.’s (2019), who reported a 300% increase of proline in Rosmarinus Officinalis L. caused by saline stress. With this, osmotically active organic compounds are syntethized, such as L-proline, a molecule considered osmoprotective under water stress conditions, as it helps maintain the plant growth and development (Hadiarto & Tran, 2011; Valliyodan & Nguyen, 2006), but the mechanism through which these osmolytes provide such protection is not yet fully understood (Ramanjulu & Bartels, 2002).
Foliar nutrient concentration
There was a significant difference in the concentration of N, P, K, and Ca, but not so for the Mg. The N concentration rose as the EC increased, while the opposite happened for K. In the case of P, no trend was defined by the treatment effect. Ca concentration decreased by reaching an EC of 2.0 dS/m (Table 6).
Table 6. Salinity effect on plant analysis on the nutrient content of papaya plants
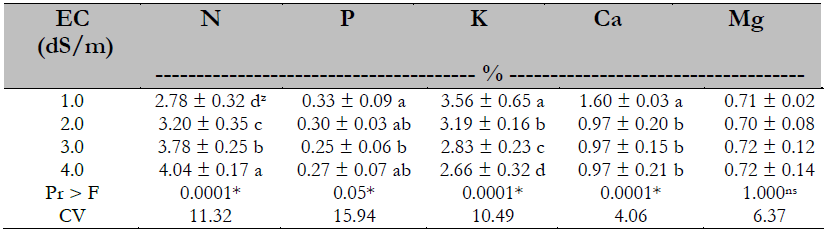
Note.CV = Coefficient of Variation; zvalues with identical letters in the same column indicate that they are statistically equal groups (Duncan’s α ≤ 0.05); ns = not significant; * = significant difference.
Source: Elaborated by the authors
Most studies indicate that nitrogen absorption is reduced under salinity conditions, although some tudies report the opposite and even no effect (Veli & Halit, 2009). The effect found in this study on foliar N concentration is contrary to what was reported by Veli and Halit (2009) in melon (Cucumis melo L.) and Al-Rawahy et al. (2009), who mentioned a reduction of 35% on N uptake in tomato (Solanum lycopersicum L. cv. Columbia) as a salinity effect. These authors attribute this behavior to a root suberization effect caused by salinity, as salinity was induced through NaCl; however, there is a similarity to what was reported by Viegas et al. (2004) in Prosopis juliflora (Sw) DC, who indicated that the percentage of nitrogen increases according to salinity increment.
There is also an increase in nitrogenous compounds as a stress response of the plant, resulting in an osmotic adjustment; being nitrogen a structural component of amino acids, proteins, and nucleic acids, a more significant amount of amino acids such as proline and choline are generated as resistance to stress (Argente et al., 2009; Becerra et al., 2019). The phosphorus concentration results in this research do not show a definite trend as to the salinity effect. In this regard, Navarro et al. (2001) mentioned that there is still controversy regarding the salinity effect on the absorption of this element.
Potassium uptake matches what Casierra et al. (2000) reported in Solamun quitoense L., Chen et al. (2001) in Populus euphratica Oliv., Khoshgoftarmanesh and Naeini (2008) in olive, and others who reported that by increasing salinity, the concentration of foliar K decreased. This behavior is related to the effect caused by decreasing salinity on density and stomatal openness, reducing the perspiration process and, therefore, the absorption of K, as indicated by Baligar (1985); where there is no root competition between plants, 97% absorption of K occurs by the mass flow route. The same is true for calcium, another nutrient dependent on mass flow for its uptake. In magnesium uptake, Parés and Basso (2013) did not find effects of salinity either in a range of 0.001 to 8.0 dS/m.
Conclusions
Steiner nutrient solution concentrations in irrigation water with EC of 4 dS/m reduced the growth of Maradol papaya plants. Plants showed changes in nutrient concentrations when exposed to irrigation with a nutrient solution greater than 2 dS/m. Maradol papaya can be considered moderately tolerant to salinity. Steiner nutrient solution with 4.0 dS/m (200%) can be used to reduce plant height and grow it in greenhouses.