1. INTRODUCTION
The importance of renewable energy sources has brought new research challenges. One of these scopes is reducing the greenhouse gases produced by the transport sector, which contributes 11.6 % and 10.7 % of global deaths due to exposure to Particulate Matter (PM) and ozone, respectively. These transport emissions mostly come from sub-Saharan Africa, Central America, parts of the Middle East and Central Asia, and Southeast Asia [1]. Nevertheless, given that flex fuels have increased their market share worldwide, there is a higher demand for renewable green fuels. Therefore, new ethanol production methods are required to enhance their capability to replace petrol fuels and compete against petrol prices.
Tropical crops (e.g. sugar cane) have a restricted use worldwide due to their high-water requirements. Hence, the low-water requirement of amylaceous crops becomes an advantage in countries with a climate that changes throughout the year. For instance, Dioscorea sp. (yam) requires a lower water application (888 mm) compared to Manihot esculenta (cassava) and corn (1492 and 1017 mm, respectively) [2], [3], [4]. Moreover, Dioscorea sp. varieties with low moisture content would be suitable for prolonged tuber storage and more efficient for industrial processing.
In this regard, Dioscorea rotundata is the Dioscorea sp. variety with the lowest moisture content (58.18 ± 1.22 % w/w) and the highest starch concentration (85.51 ± 1.21 % w/w) in dry basis [5]. Also, its starch concentration surpasses that of corn and cassava in a dry basis (78 % and 35 % w/w, respectively), making it a potential feedstock for ethanol production [6].
However, ethanol production from amylaceous crops requires a starch hydrolysis process before fermentation, which increases direct costs. This has been observed in feedstocks such as corn, with 35 % higher production costs per liter of ethanol than sugarcane [7].
Consequently, evaluating new amylaceous feedstocks could minimize said costs, thus leading to a smaller difference between petrol fuel and flex fuel.
The biotechnological route could reduce energy requirements and fix CO2 through farming growth. Saccharomyces cerevisiae is the most widely used fermenting yeast thanks to some of its strains resistant to high temperatures and ethanol. Bioethanol can be produced via fermentation using two methods: Separate Hydrolysis and Fermentation (SHF) and Simultaneous Saccharification and Fermentation (SSF). Both employ starch to produce ethanol and comprise the same phases. In the first phase, enzymatic hydrolysis is applied employing α-amylase and glucoamylase enzymes for starch degradation, thus reducing polysaccharides to monosaccharides and some disaccharides used in further fermentation.
Nevertheless, SHF lasts over 72 h, while SSF only requires 36 h [8]. This time difference between both methods is attributable to the time required for hydrolysis. In SSF, enzymatic hydrolysis simultaneously occurs with fermentation (prior enzymatic pretreatment of starch), while, in SHF, starch is completely hydrolyzed before fermentation. Different raw materials have been used for glucose and ethanol production from tubers.
In [9], glucose production from cassava was studied, and a concentration of 9.1 % w/w of remaining starch was obtained after 2 h of enzymatic hydrolysis with α-amylase. Some authors have already used tubers in SHF and SSF and stressed the benefits of SSF over those of SHF. For instance, starch has been hydrolyzed and fermented via SSF with cassava flour and Saccharomyces cerevisiae, thus obtaining 200.48 g/L of Total Reducing Sugars (TRS) with 79 % of hydrolyzed starch after heating the system to 86 °C at 500 rpm, as in [10]. In this latter study, the authors managed to reduce the time for hydrolysis to 1 h, including heating time (10 min at a constant temperature) and cooling time (at 37 °C). In addition, they obtained an ethanol concentration of 115.50 g/L in the SSF method at 37 °C and 641.4 rpm after 72 h of fermentation. Other researchers have applied SSF after liquefaction. As a result, they have obtained 47.2 % of hydrolyzed starch after 2.5 h and an ethanol concentration of 47.2 ± 2.1 g/L and reached 78 % of fermentation yield and 1.33 g/L h of ethanol productivity using α-amylase enzyme (Liquozyme SC®) and Saccharomyces cerevisiae (Instant Yeast®) [8].
Despite its low-water requirement and production costs, Dioscorea sp. has been little studied. This yam is mainly produced in tropical countries, with Nigeria, Ghana, and Cote d’Ivoire as the leading producing countries around the world [11]. Colombia-the second producer of Dioscorea sp. in Latin America and the Caribbean [11], but the country with the highest productivity worldwide [12]- can open new paths to help lower-middle economy countries grow around the modern use of this type of yam, in which smallholder farmers are involved [13]. Furthermore, the high starch concentration (close to 90 % w/w in a dry basis) of Dioscorea sp. makes it possible to produce high concentrations of ethanol provided that enzymatic hydrolysis occurs entirely [3].
In some studies, Dioscorea sp. has been employed to produce glucose and ethanol. In particular, Dioscorea alata, Dioscorea esculenta, and Dioscorea hispida have been analyzed to evaluate their potential for ethanol production. According to the results of these studies, 65.95 g/L, 64.17 g/L, and 59.19 g/L of TRS were obtained, respectively, after enzymatic starch digestion with 0.1 % w/w of α-amylase [14]. Additionally, studies that used Dioscorea sansibarensis reported 68 % w/w of total carbohydrates in a dry basis after enzymatic hydrolysis and an ethanol concentration of 56 g/L at the end of batch fermentation, as in [15]. Other works achieved an ethanol production of 425 L/ton from Dioscorea bulbifera starch and 251 L/ton from Dioscorea bulbifera peels [16].
Dioscorea rotundata is the second of three main Dioscorea sp. varieties produced in Colombia [12]. Some authors have studied its use in ethanol production via SHF and reported a concentration of 30.6 % w/w of TRS after enzymatic hydrolysis and 0.25 g/g of product-substrate yield (YP/S) after five days of fermentation with Saccharomyces cerevisiae [17].
However, a higher hydrolysis yield was achieved after 28 h of enzymatic hydrolysis (95 % of hydrolyzed starch) and 0.47 g/g of YP/S were obtained after 52 h of fermentation with Saccharomyces cerevisiae, as in [18].
In view of the above, the main purpose of this study is to evaluate TRS concentration and ethanol yield from Dioscorea rotundata via enzymatic hydrolysis and SSF, respectively, using Saccharomyces bayanus as a potential substitute for the well-known Saccharomyces cerevisiae.
2. MATERIALS AND METHODS
2.1 Dioscorea rotundata and experiment preparation
Dioscorea rotundata peels were cut and removed, and the peeled yam was mashed. Subsequently, each sample was prepared using different yam concentrations (10 %, 12.5 %, 15 %, and 18 % w/w) in 250 mL of distilled water.
2.2 Gelatinization and enzymatic treatment
Samples were gelatinized for 60 min on a 10-place heater with a stirring speed of 250 rpm and at 68 °C. The temperature was increased to 90 °C for the liquefaction phase, while the stirring speed was maintained. After adding 0.007 mL of α-amylase (Amiltex 35 NP) per yam gram, the samples were rested for 40 min. Finally, they were subjected to saccharification by adding glucoamylase (Naturalzyme GA 300 L) in a ratio of 0.004 mL per yam gram and rested for 20 min at 60 °C. Subsequently, temperature was reduced to 30 °C for the SSF method to simultaneously continue with the saccharification process during the fermentation stage.
2.3 Simultaneous saccharification and fermentation
The Simultaneous Saccharification and Fermentation (SSF) method was used to make the most of its low-energy requirements and time savings. For this process, 2.5 g/L of Saccharomyces bayanus (SafCider-Fermentis®) was added to each experiment after its hydration with ten times its weight in a water mass at 35 °C for 15 min. The temperature was reduced to 30 °C, the pH was adjusted to 5 with HCl 1 N, and the stirring speed was set to 250 rpm for 27 h. Samples of each experiment were taken at the beginning and after 15, 18, 22, 25, and 27 h.
2.4 TRS and ethanol quantitation
The 3,5-dinitrosalicylic acid (DNS) technique [19] was employed to measure TRS concentration with a Shimadzu’s UV-1800 spectrophotometer at 540 nm. A calibration curve was fitted using glucose at concentrations from 0.5 to 2 g/L.
Ethanol concentration was analyzed by means of High-Performance Liquid Chromatography (HPLC) in a Dionex UltiMate 3000 (Thermo Scientific, USA) equipped with a Shodex RI-101 detector (Showa Denko K.K., Japan) and a Shodex SH1821 column operating at 75 °C with 0.5 mM H2SO4 as mobile phase (0.6 mL min-1).
The hydrolysis results were evaluated based on the hydrolysis yield (1). The theoretical moisture was 58 % w/w, value that was used to calculate the theoretical starch mass concentration [5]
Where
Y H = Hydrolysis yield.
c f = Final starch concentration.
c t = Theoretical starch concentration.
The SSF results were assessed based on the product-substrate yield (2), the fermentation yield (3), and the ethanol productivity (4) from the product and substrate concentrations (denoted as P and S, respectively).
Where
Y P/S = Product-substrate yield.
Y F = Fermentation yield.
P f = Final product concentration.
P o = Initial product concentration.
S f = Final substrate concentration.
So = Initial substrate concentration.
t = Time.
3. RESULTS AND DISCUSSION
3.1 Enzymatic treatment results
The hydrolysis yield per each yam concentration was calculated considering the theoretical starch mass content (Figure 1). According to the results, the samples with yam concentrations of 10 % and 15 % w/w exhibited the highest yield (almost 90 % of starch hydrolyzed to reducing sugars before SSF). Nevertheless, the TRS content in the sample with a yam concentration of 15 % w/w (111.2 ± 3 g/L) was 58.9 % greater than that of the sample with a yam concentration of 10 % w/w (69.9 ± 4.5 g/L).
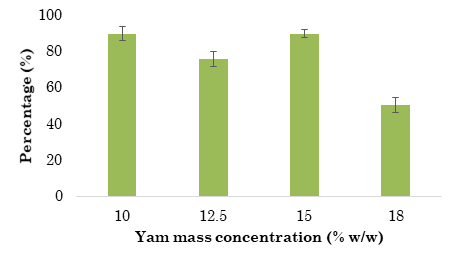
Source: Authors’ own work.
Figure 1 Hydrolysis yield per Dioscorea rotundata mass concentration. Data represent the average of triplicates, and error bars correspond to the Standard Deviation (SD)
This hydrolysis yield is consistent with the results in [9]. In said study, the authors reported a concentration of 91 % w/w of hydrolyzed starch after cassava flour was hydrolyzed with α-amylase for 2 h (one hour more than the time employed in this study). Also, this yield doubled those reported in [8], [20], and [21] from cassava starch (47.2 %, 42.4 %, and 76.8 %, respectively). Additionally, the sample with a yam concentration of 10 % w/w achieved a TRS concentration similar to that obtained in [14] after the enzymatic hydrolysis of 10 % w/v of Dioscorea alata, Dioscorea esculenta, and Dioscorea hispida.
Moreover, our results can be compared to those obtained with other Andean tubers (i.e., cassava, potato, and sweet potato). For instance, the authors in [20] evaluated the optimal starch concentration of the three tubers mentioned above for enzymatic hydrolysis and ethanol production via Sequential Saccharification and Fermentation (SeqSF) and SSF. Their results are similar to those of the Dioscorea rotundata starch obtained in this study. However, the reducing sugar concentration after saccharification was higher for Dioscorea rotundata (90 ± 4 % of starch hydrolysis yield versus 52, 56, and 58 % for cassava, potato, and sweet potato, respectively). Furthermore, the hydrolysis yield reported here (90 ±2 % of hydrolysis yield and 111.2 g/L of TRS) is higher than that of all starchy sources evaluated in [20] for trays with a yam concentration of 15 % w/w (49, 50, and 52 % of hydrolysis yield and 83, 85, and 78 g/L of TRS).
Compared to very high gravity systems, our results are still better than the hydrolysis yields of rye starch (25 and 28 % w/w) reported in [22]. For all cases, the results were optimized under the conditions evaluated in this study.
The ANOVA results showed a statistical significance (p ≤ 0.05) between initial yam concentration and TRS production after hydrolysis. Consequently, initial yam concentration affects TRS concentration after hydrolysis.
3.2 SSF results
TRS concentration was measured throughout the SSF process. As shown in the TRS consumption plot (Figure 2), a stabilization is observed from hour 22, with TRS concentrations close to zero for each system, as stated in [23], [22], and [24]. Hence, TRS consumption was 50 % faster than the results obtained in [18], where the TRS concentration was close to zero after 50 h of fermentation, applying SHF from Dioscorea rotundata. Furthermore, TRS consumption was 30 % faster than that reported in [8], in which authors achieved a TRS concentration close to zero after 27 h, applying SSF from cassava flour. In the first 24 h, this behavior suggests that rapid TRS reduction is mainly caused by cell growth, as proposed in [22]
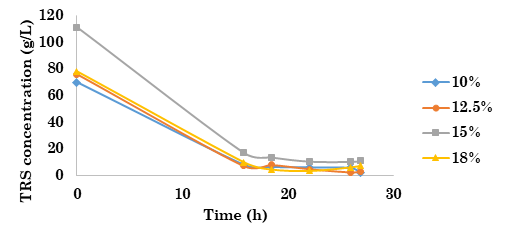
Source: Authors’ own work.
Figure 2 TRS concentration in the SSF process for the mass load of Dioscorea rotundata over time
According to the ANOVA test results, there is no statistical significance (p ≥ 0.05) between remaining TRS (at the end of SSF) and initial yam concentration. Conversely, remaining TRS depends on available TRS at the beginning of SSF (p ≤ 0.05).
The highest ethanol productivity and YP/S were observed in the system with a yam concentration of 10 % w/w (Table 1). By comparison, in the systems with a yam concentration from 12.5 % to 18 % w/w, the samples’ productivity showed a positive linear trend. However, if these results are compared to those obtained with other tubers, YP/S was 84.5% lower than the results reported in [8] from cassava flour fermentation via SSF. Moreover, the fermentation yield was lower than that obtained in [23] (82, 79, and close to 95 %) from cassava, potato, and sweet potato starch.
Regarding final ethanol concentration, our results are marginal if compared with the fermentation results reported in [20] (27.4 g/L of final ethanol concentration and 57.8 % of fermentation yield) and [22] (94.6 ± 1.8 g/L of ethanol concentration and 84.2 % of fermentation yield after 72 h of fermentation).
Despite the low fermentation performance, the resulting volumetric ethanol concentration (Table 1) was similar to that obtained in [25], from 78 g/L of initial TRS, using Saccharomyces bayanus after 24 h of fermentation. Saccharomyces bayanus achieved its maximum ethanol production ratio from 48 to 72 h of fermentation. This behavior is supported by the results reported in [26], where the authors obtained an ethanol concentration similar to that in [25] after 36 h of fermentation via SSF using hydrolyzed sugarcane bagasse.
Nevertheless, the obtained ethanol productivity and yield confirm that the yeast strain used in this study is not feasible for fast ethanol production via SSF. Therefore, further improvements in terms of increased ethanol yields are necessary to achieve a viable economic process using this potential feedstock that has proven to have a better hydrolysis phase than other tubers.
Although the authors in [23] stress the benefits of SSF over those of SeqSF in terms of fermentation yield and time, integrating Simultaneous Saccharification and Co-Fermentation (SSCF) and Fed-Batch reactor configuration could become an alternative to improve the economic feasibility of ethanol production, as proposed in [24] with a very high gravity process.
4. CONCLUSIONS
Dioscorea rotundata has a great potential as raw material for lactic and alcoholic fermentation due to its high reducing sugar concentration after hydrolysis. However, Saccharomyces bayanus is not suitable to replace Saccharomyces cerevisiae in ethanol production via SSF due to its low ethanol productivity in the first 24 h of fermentation.