Introduction
According to the World Health Organization (WHO), at the beginning of the second decade of this century, annual deaths from cancer reached 10 million with 400,000 diagnoses in the child population (WHO, 2022) and a 60% increase is expected for the next 20 years. This scenario implies an important challenge for science and technology in the fields of the prevention, early diagnosis, and treatment of the disease. In this sense, emerging nanoscale technologies can contribute to addressing this severe public health problem.
The development of new methods for nanoparticle synthesis that control their material, size, shape, and composition has opened the way for the fifth generation of nanomaterials (Parak, 2011; González et al., 2011) enabling the search and consolidation of viable solutions. To reach a plateau in the adoption of these emerging alternatives, a sufficient level of control of the following aspects should be achieved: i) efficient transport and distribution of the nanoparticles in the biological medium of interest; ii) cellular internalization (if necessary), which implies understanding sufficiently the cell-nanoparticle interaction; iii) the transport capacity and pulsatile or sustained release of pharmacological agents including, in some cases, controlled sequential delivery of multiple drugs for co-administration; iv) in some situations, an effective thermal and optical response of the nanoparticle is necessary, and v) biocompatibility of the nanostructure material. Compliance with these aspects will allow for improving pharmacological options, reducing side effects, and reducing the doses required by modulating selective toxicity.
Besides considering the cell type, the internalization, toxicity, mobility, and distribution of nanoparticles in cells of interest will depend on factors such as size, shape, composition, aggregation, charge, and surface functionalization, factors that are determined by the synthesis method used. The many studies reporting on the correlations between these factors and the cellular response open a path to important findings and useful information to understand the underlying mechanisms in cell-nanoparticle interactions (Price et al., 2019; Yue et al., 2018; Niaz et al., 2022; Augustine et al., 2020), which will allow the identification of the criteria and conditions required in the design of highly efficient and functional nanoparticle-based systems for use in diagnostic and treatment tasks in the holistic context of theragnosis (Mitchell et al., 2020).
Among the great variety of nanoparticles offered by the synthetic methods developed to date (Kim et al., 2009), gold nanoparticles stand out for their outstanding physical and chemical properties. They have an optimal thermal response and optical cross-section, which is related to the area of the nanoparticle that can intercept the incident light flux producing absorption (quantified by the C b coefficient) and/or scattering (quantified by the C c coefficient). The optical cross-section depends on the size and geometry of the nanoparticle and can be tuned allowing defined displacements of the surface plasmon resonances. Additionally, gold nanoparticles have low reactivity and are favorable to surface modification and conjugation, an essential condition for programming and controlling functions. This positions such nanostructures as strategic for research in thermotherapy (Vines et al., 2019), pharmacokinetics, and pharmacodynamics, specifically in transport, controlled drug release, and diagnostics (Carnova et al., 2016). Regarding health risk, there is still not enough information and understanding of the processes and causal factors to allow for a conclusive and consensual assessment.
From research carried out with hollow and porous gold nanoparticles, the use of these entities is valued for the design of devices and multifunction systems for medical applications that comply with the previously mentioned requirements (Qui et al., 2020; Qui et al., 2021). On the other hand, using the external surface of the nanoparticle within the scope of volume and surface control and programming (González, 2016) it is possible to develop complex multifunctional platforms that allow, among other options, drug delivery with a controlled sequential release where a first drug sensitizes the tumor environment (e.g., tumor priming) while the second one achieves therapeutic success. Combinatorial sequential therapy is, without a doubt, one of the promising options to improve the quality of life and treatment of cancer patients.
Faced with the difficulties of pharmacological treatments due to the resistance of cancer to multidrug therapy and the barriers imposed by the tumor environment, methodologies for sequential delivery with synergistic effects using the advances in nanomaterials science and engineering have aroused a growing interest in alternatives to face this challenge (Xu et al., 2015; Morton et al., 2014; Yao et al., 2020). Interesting proposals have already been investigated for particulate systems with the sequential capacity to release molecular agents involving the volume of the particle, the surface, or a molecular matrix, generally polymeric. Zhao et al. (2020) have reported the release by diffusion of the antibacterial agent methylisothiazolinone from titanium dioxide microspheres as a molecular model taking advantage of the hydrogen bonding capabilities with titanium dioxide and the rc-rc arrangements between the molecules used. The sequential release of molecular agents from the surface of hollow silica nanoparticles has also been reported and photothermally activated cRGD peptide-modified nanocarriers for dual drug delivery with gold nanorods have been designed (Zhu et al., 2019).
Taking advantage of the morphology of double-walled nanoboxes that configure a nanochannel and a central cavity, a multifunctional platform for sequential delivery of molecular agents is proposed. This nanostructure is also used as a carrier and opto-thermal nanoantenna. The surface was programmed with molecular agents allowing for the recognition of the target of interest, the internalization, and the control of cargo release, among other features. For the dimensions, shape, and composition of the nanoparticles obtained, the sequential release kinetic response of two molecules of interest was evaluated through a computational simulation. The results derived from this research aim at evaluating the diffusive dynamics of molecular agents at a nanoscale level within a pharmacological context acknowledging the limitations that its implementation implies, as there are still some issues to be addressed.
Materials and methods
For the nanobox synthesis, the following reagents were used: silver nitrate >99%, gold hydrochloride 99.99%, polyvinylpyrrolidone with a molecular weight of 55K, cetyltri-methylammonium bromide (CTAB), ascorbic acid 99%, ethylene glycol 99.8%, and sodium sulfide nonahydrate 99.9%. All reagents were purchased from Sigma Aldrich and used as received. The synthesis was performed following the method developed to control size, shape, and composition reported in González et al. (2011). For the synthesis of double-walled nanoboxes at room temperature, we started with the dispersion of completely washed silver nanocubes in milli-Q water at a 10-3 nM concentration synthesized following the method proposed in Fievet et al. (1989). At room temperature and permanent stirring at ~ 200 rpm, CTAB was added to this colloid at 1.4x10-2 M followed by ascorbic acid at 1.4x10-4 M and gold hydrochloride 5.5x10-5 M with a flow (Q) determined as follows:
where V is the volume of gold hydrochloride loaded in the micropump in μL. The width of the wall was controlled by modifying the CTAB and ascorbic acid concentrations and the reaction time. The size of the nanoboxes was determined by the size of the silver templates used, which can be controlled during their synthesis.
Results and discussion
DWSPNb structure and composition of
As a result of the structural, geometric, and compositional control of the nanoparticles obtained by galvanic replacement and the Kirkendall effect, we obtained the colloidal dispersion of double-walled hollow cubic complex nanoparticles (Figure 1A). The edge length of the nanoboxes obtained for the design of this platform was 49±5 nm with a monodispersity close to 90% (Figure 1B).
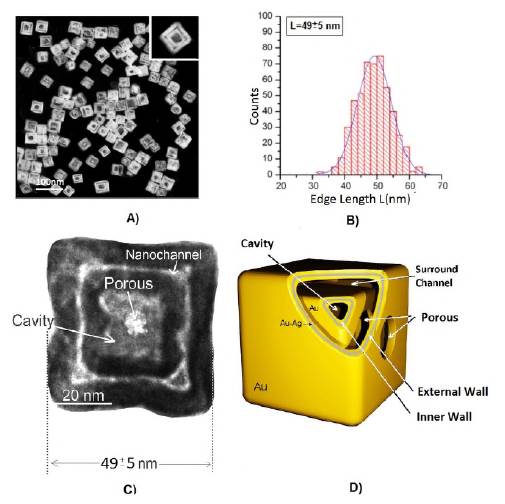
Figure 1 A) Image taken with transmission electron microscope of synthesized nanoboxes. B) Size distribution of the nanoboxes. C) Transmission electron microscope photograph of a DWSPNb, in which the main dimensions and structure are indicated. D) Three-dimensional geo-metric representation of the nanobox
The nanoparticle was formed by the following structures: i) A cubic nanochannel formed by the walls of the nanobox. The average distance between the walls was approximately 2.5+0.4 nm. This distance can be increased by modifying times and reagent concentration in the synthesis process and boxes with 5 nm distances can be obtained; ii) a cavity in the center of the nanobox, and iii) an entrance pore to the nanochannel and the cavity of 7+2 nm in diameter. The dimensions of the nanochannel and the entrance pore can be controlled during the nanoparticle formation process. The surfaces of the walls were made of 100% gold while their inside is a gold-silver alloy with proportions that can be controlled during the synthesis process (Figure 1C-D).
One of the main advantages of DWSPNb made with gold compared to non-metallic nanoparticles is their optical and thermal properties. The fact that they are made of gold makes them useful as a thermo-optical nanoantenna for thermotherapy and diagnosis, as well as for the activation of polymers and the mobility of molecular agents contained in the nanoparticle. The therapeutic uses of laser radiation for thermal and optical excitation require the nanoparticle to have the surface plasmon resonance peak within the wavelength range belonging to the first water window. For this 650 nm to 900 nm interval, the biological environment is transparent to radiation but not for nanoparticles, which absorb with maximum efficiency in this region. Figure 2A shows the absorption spectrum as a function of wavelength for the nanoboxes with a plasmon resonance peak at 680 nm. As can be seen, the resonance peak belongs to the bandwidth of the first water window, which makes these nanoparticles an exceptional option for multifunctional platform design.
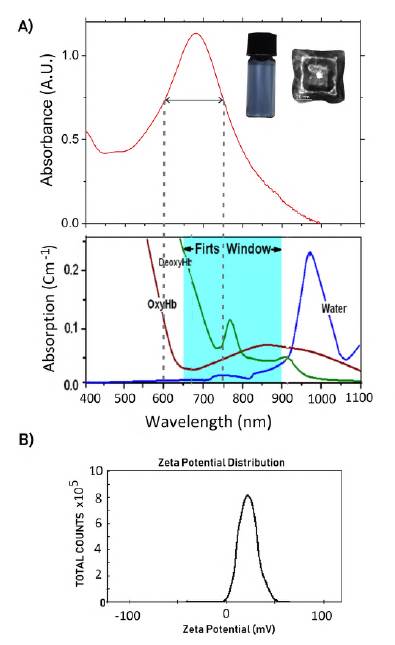
Figure 2 A) Absorption spectrum for the DWSPNb. As can be seen, the surface plasmon resonance peak belongs to the first water window for wavelengths between 650-900 nm. The blue color exhibited by the colloidal dispersion of the DWSPNb is shown. B) Z-potential for the synthesized nanoboxes. A value of +23.5 mV is recorded
Depending on the chemical agents used in the nanoboxes synthesis, the surfaces are characterized by a positive Z-potential with values that can be tuned as a function of surfactant concentration. The graph in figure 2B shows the Z-potential value for the nanoboxes synthesized in this study, which corresponds to +23.5 mV.
The platform for controlled release
The general scheme of the multifunctional platform for the sequential space-time release of therapeutic molecules proposed in this study is shown in figure 3. The core of the system consisted of the double-walled hollow cubic gold nanoparticle. Since the nanobox had pores crossing both walls whose diameter can be controlled during the synthesis process, the therapeutic molecules TM1 and TM2 chosen for the computational evaluation of the platform will be confined in the central cavity and the two-dimensional cavity or nano-channel that forms the two walls of the nanobox, before their release. Most drugs used for pharmacological treatment in cancer classified as "small drugs" have a 0.4 to 2 nm diameter.
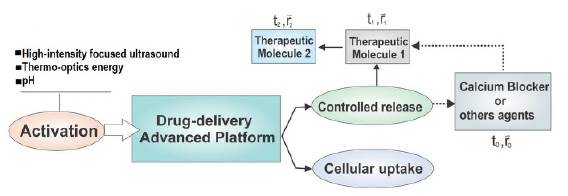
Figure 3 General scheme of the platform for sequential release controlled in time and place of therapeutic molecules.
The exit door for the molecules of interest was covered by a polymer (goalkeeper) that can be activated by near-infrared (NIR) light, high-intensity focused ultrasound (HIFU), or by changes in the environment pH intended to prevent or allow, as required, the release of the molecules through the nanobox pore. When the pore opened due to the activation of the polymer used, it was clear that the release of the molecules was sequential.
HIFU is a therapeutic technique for cancer treatment that uses the energy of focused ultrasound. It is minimally invasive and given that ultrasound is non-ionizing, it can be performed several times without significant side effects on the tissues. As has been reported (Sadeghi et al., 2019), the absorption rate of acoustic energy is optimized with the use of gold nanoparticles and part of this energy heats the nanoparticles. For gold nanocages, controlled drug delivery using HIFU for activation of thermally responsive polymers has already been tested (Li et al., 2011).
Here the nanobox surface was functionalized with the corresponding antigen for the recognition of the target, in this case, the tumor cell. Besides, calcium blockers or other molecules with the ability to improve cell internalization and other functions determined by the type of cell and biological environment for which the platform is configured can be attached.
The following advantages of this nanoplatform stand out: It provides an interesting option for a sequential and controlled delivery using nanochannels and pores. It can be programmed in dispersion mode for use in medical imaging or as a thermotherapeutic agent and activator of thermosensitive polymers. During the DWNBx synthesis process, it is possible to program the pore size, the channel width, and the wall thickness to modulate the thermal, optical, and drug delivery properties. Among the different structures offering sequential release alternatives, nanoboxes structural morphology allows for the implementation of nanofluidics, which is not an option with most carriers for sequential delivery and is one of the main differentiating factors of these nanoparticles. Besides, the volume of the nanochannel, the nanopores, and the cavity, and their structural distribution, turn the nanostructure into a nanoreactor for chemical reactions controlled at the nanoscale offering an interesting field of research for other types of functions besides those proposed here.
Nanochannel and nanopores
Nanoflows are usually defined as fluid flows at the nanometer scale. The devices in which they generally transit are nanochannels and nanopores, which have been widely studied for their potential applications in catalysis, sensing, drug delivery, molecular transport, selective molecular separation, molecular gates, nanofiltration, and DNA sequencing, among others (Thomas et al., 2001; Han et al., 2008). They exist in biological systems or can be artificially manufactured with diameters ranging from the Armstrong scale to the nanometer scale (Karniadakis et al., 2005). Fluidic nanochannels 1-2 nm in diameter have been used to investigate molecular and ionic transport in confined liquids. Holt et al. (2006) reported the fabrication of aligned carbon nanotubes with diameters of less than 2 nanometers for water flow measurements (Holt et al., 2006). On the other hand, using electroless deposition, nanochannels with gold-polymer composites and diameters less than 5 nm that allow functional modification of the gold inner surface have been manufactured (Harrell et al., 2004).
As reported in Ahuja et al. (2009), the porosity for drug delivery can be classified depending on the size as microporosity when the width is less than 2 nm, mesoporosity for a 2 to 50 nm width (according to the IUPAC definition, these pores give rise to capillary condensation phenomena), and macroporosity for width over 50 nm. When the pore connects different parts of the interior with the surroundings of the structure, it is often referred to as a transport pore. According to this classification, the DWNBx connects the cavity, the nanochannel, and the outside through transport pores. For pores with dimensions comparable to the molecules passing through them, the diffusion coefficient is lower than in bulk. For cylindrical pores, hindered diffusion models have already been developed for the study of these transport processes (Malone et al., 1978).
The Péclet number of nanofluidic systems, defined as the ratio of the rate of advective transport to the mass rate of diffusion, ranges from 10-6 to 1 suggesting an important role of molecular diffusion with an important mark of anisotropy. Diffusion in nanochannels and nanopores does not strictly obey Fick's law due to the spatial confinement that accentuates the interaction of the flow with the walls.
The DWNBx proposed here as a potential sequential delivery nanoplatform are nanoparticles that morphologically exhibit transport pores and structures that can be considered as nanochannels. The pores can be of cylindrical geometry. The diameter of the pores plays a very important role in the loading and unloading of the molecular agents of interest. If it is assumed that TM1 is stored in the cavity and TM2 in the nanochannel, and these two molecular entities are of similar sizes, the protocol in the process of loading is much more complex than that required with TM1 larger than TM2 because the diameter of the entrance pores to the cavity is greater than the entrance width to the nanochannel, which facilitates selective loading by size.
Molecular diffusion in the nanoboxes
The molecular agent transiting the nanochannel is drastically confined to a scale that produces transport behaviors subject to loss of homogeneity and fluctuations in the density and viscosity of the solvent (Thomas et al., 2009). The experimental results reported for molecular diffusion in channels with nanoscale confinement have enabled the evaluation of the existing theoretical models, which are insufficient to explain transport at these scales, an aspect that corroborates the great limitations of our knowledge of the diffusive theory at the nanoscale (Bruno et al., 2018).
Once synthesized, the nanoparticle surfaces preserve the distribution of electrical charge and potential gradients caused, among other factors, by the ionization of surface groups, the substitution of surface ions, and the specific absorption of ions. Consequently, an electric field must be produced on the surface, which, although weakened with distance, generates a layer of counterions called the Stern layer. As the distance from the surface increases, the ionic concentration decreases, producing a second layer that is called diffuse. The model incorporating these two layers is called the double electrical layer. The potential i|/ produced by the ionic charge density can be calculated from the Poisson and the Boltzmann equations known as the Poisson-Boltzmann approach (González et al., 2015):
where p corresponds to the ionic charge density, ε is the dielectric constant of the solution, e the elementary electric charge, z i . the charge number of the ith ion, K the Boltzman constant, and T the absolute temperature. Equation (3) can be approximated to a linear equation for low-intensity potentials, below 25 mV, which holds for the synthesized nanoboxes:
with a solution of the form:
where k corresponds to the inverse of the diffuse layer distance known as the Debye length.
If the Debye length is greater than 1/10 of the channel width, as is the case for the nanochannel under consideration, the overlapping of the electrical double layer occurs leading to electrostatic confinement for the solute transiting the channel. Since the width of the double layer is inversely proportional to the ion concentration, the Debye length decreases with increasing ion concentration. If the solute is neutral, this contribution can be considered negligible and a rigid sphere model and hydrodynamic interactions with the channel walls can be used (Bruno et al., 2018).
For modeling the release of the molecular agents TM1 and TM2, the structural morphology of the nanoboxes and the nanometer-scale spatial confinement for the mobility of molecular entities must be considered. Due to the interactions by proximity between the molecular agents and the walls of the nanochannel or nanopore, the correction of the approximations usually used for continuous homogeneous media is required. Molecular dynamics provides useful information to scale and refine the macroscopic models commonly using the finite element method. This approach is known as hierarchical multiscale modeling (Ziemys et al., 2011), which is an interesting option to develop the predictive model for the release of molecular agents TM1 and TM2 from the nanoboxes.
In molecular dynamics, the motion of particles is determined by the second law of mechanics. Interactions include bonded, electrostatic, and van der Waals forces (VDW). The forces are calculated from the intramolecular and intermolecular potential energy function E=Eintra +Eintra (Kojic et al., 2011):
where Kb, Kθ, K+ are the force constants, b0 the equilibrium values of bonds, 5 the dihedral phase, n the dihedral multiplicity, ey the van der Waals potential depth, Rmin the atom radius, and qi, qj the partial atomic charge.
To calculate the diffusivity, the mean square displacement (MSD) obtained from the Einstein relation is used:
where <r 2 (t)> is the MSD, D the diffusion coefficient, and t the duration. MDS can be computed from the following expression:
where N is the number of equivalent particles and d the dimensionality of the MSD. In Maginn et al. (2019) details of the MSD calculation are reported.
If a two-dimensional section of one of the faces of the nanochannel is taken, from a calculation made for 20 trajectories, using a 10 ps time window, the diffusion coefficient is obtained as a function of the distance to the wall of the nanochannel for a given concentration (Ziemys et al., 2010). Here, the MDAnalysis MSD module corresponding to an object-oriented python toolkit was used (Calandrini et al., 2011; Buyl et al., 2018) and the results obtained for the doxorubicin molecule at a concentration of 1M are shown in figure 4. With this approach, the molecules would present zero diffusion for distances less than 0.4 nm from the wall. Since the channel has a 2 nm separation between the walls, the diffusion that should be taken to model the release would be approximately equal to 0.36 x10-9 m2/s if transit through the middle of the channel is assumed.
Thermo-opticalresponse of the DWSPNb
Due to the composition of the DWSPNb: gold on the outer walls and gold/silver alloy on the inside, its opto-thermal response is of great interest for controlled activation of the molecular agents contained, as well as for its complementary thermos-therapeutic action. As has already been reported for hollow gold nanoparticles with a gold-silver alloy inside (González, 2016), the thickness of the wall drastically determines the plasmon resonance peak. As the wall thickness increases, the surface plasmon peak experiences a blue shift. The relationship between absorption and scattering is determined by the length of the edge so that by decreasing length, absorption increases over scattering.
On the other hand, the laser energy excites the electrons and produces a hot electron distribution. The increase in the electronic temperature modifies the occupation of the electronic states close to the Fermi level and produces a broadening of the plasmonic band. This behavior depends on the laser intensity, wavelength, and gold absorption. The electrons reach equilibrium with the lattice through electron-phonon couplings. In time scales close to 100 ps, the heat is dissipated until thermal equilibrium with the surroundings is reached (Mahmound et al., 2010).
For the DWSPNb, the modeling was performed by the finite element method (Cao et al., 2019) for a wavelength equal to 650 nm, which corresponds to that in the surface plasmon resonance bandwidth for the nanoboxes and belongs to the first water window as indicated in figure 2A where the laser radiation used for thermotherapy or goalkeeper polymer activation, makes its attenuation by the biological environment much lower than that of the boxes and, consequently, the absorption there allows for its thermal action.
According to the results reported here (Figure 5), the distribution of the electric field shows hot zones in the cavities whose intensity in the central cavity is greater than the nanochannel by approximately a factor of 2.3. This behavior is explained by the type of geometry offered by cubic cages where plasmonic couplings production depends on the distance between the faces, as reported for hollow gold nanoparticles (Mahmound et al., 2010; Azis et al., 2016; González, 2016). On the other hand, the thickness of the wall was decisive in the interwall coupling between the surface plasmon fields of the internal and external surfaces, respectively.
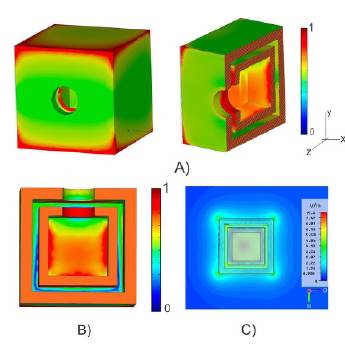
Figure 5 A-B) Distribution of the electric field for an electromagnetic wave with a wavelength of 650 nm incident in the z-direction. The box is shown in 3D, as well as a cross-section and projection in the z-y plane. C) Contour map of the electric field of a wave incident in the z-direction, which coincides with the orientation of the nanopore. The cross-section corresponding to the x-y plane is shown.
The distribution control of the electric field and, consequently, the thermal response of the nanoboxes enabled the introduction of an input variable to activate and tune the release of the two molecular entities loaded in the cavity and the nanochannel. A thermosensitive polymer can be thermally excited to open or close the nanopore according to time and place requirements. On the other hand, the photothermal effect is related to the ability to transduce optical energy into thermal energy. When exposed to optical radiation, the DWSPNb converts the absorbed photons into phonons and produces an increase in the temperature of the crystalline structure that makes up the nanoparticle. The dissipated heat can be used to damage tumor cells if hyperthermic temperatures above 42oC are reached. The capacity of DWSPNb for sequential drug delivery, the opto-thermal transduction for diagnostic imaging, and thermotherapy represent a portfolio of the options offered by this multifunctional platform configured to facilitate theragnosis viability.
Combinatorial for sequential release
One of the first-line combinations given its high efficacy has been the sequential administration of paclitaxel (PTX) and doxorubicin (DOX) (Xu et al., 2015). Pretreatment with the first drug lowers interstitial fluid pressure, improves oxygenation, and increases interstitial space, which prepares the tumor for the release and greater efficacy of the second drug (Cho etal., 2011). PTX is classified as one of the most effective antimicrotubular therapeutic agents for the treatment of different types of cancer. It has a highly complex chemical structure made up of eleven stereocenters but one of its limitations is the low solubility in water (~0.4x10-6 g/mL) demanding the use of a mixture of dehydrated ethanol with Cremophor® (Taxol) that produces significant side effects. To improve the water solubility of PTX, numerous strategies have already been reported, among which the use of amphiphilic solubilizing polymers such as 2-methacryloloxyethyl phosphorylchloline (MPC) stands out as it improves water solubility with no adverse effects (Konno et al., 2003). On the other hand, cyclodextrins increase the solubility of PTX, as well as its bioactivity (Hamada et al., 2006), while the use of polypeptides (Poly-L-lysine, Poly-y -glutamic acid, or collagen) increases the solubilization and stability of PTX in water 2700 times more than the use of Dextran-PTX conjugates (solubility ~ 80 mg/ml) and 14 times more than Taxol (Nakamura et al., 2010). It is also possible to increase the solubility of PTX in water with phosphate esters, thus improving antitumor activity and reducing cytotoxicity. For loading the drugs in the DWSPNb, specifically the PTX, its solubility in water may be required, which can be solved with the appropriate conjugation.
Gold nanoparticles have already been used in the transport and delivery of PTX by binding the PTX to functionalized phenolic terminations on the nanoparticle surfaces using hexamethylene glycol as a ligand. For a single 4 nm diameter particle, an approximate binding number of 70 paclitaxel molecules has been determined (Gibson et al., 2007). In the case of a DWSPNb, the internal cavity would be able to house at least 1.132 PTX molecules.
In the loading process, the PTX (in a soluble solvent) penetrates the central cavity of the box through the 7 nm diameter pore. Since the diameter of the PTX+ solubilizer polymer is greater than 2 nm, there is no limitation for this input. However, it cannot enter the surrounding channel whose distance for entry is close to 2 nm, which is satisfied for cisplatin, whose required value for entry is 0.4 nm, or for a DOX molecule, with a required value of at least 1.5 nm.
DOX is an anticancer therapeutic molecule used to treat solid tumors, lymphomas, and leukemias. The use of this drug is restricted by its potential cardiotoxic effects and limited distribution in solid tumors, aspects that require drastic improvement in dose optimization and administration site precision. Unlike DOX, which is hydrophobic and insoluble in water, the molecule doxorubicin hydrochloride (DOX-HCl) is a hydrophilic molecule with high solubility in water, close to 50 mg/ml, and a size of around 1.4 nm. Due to its size, this molecule can enter the surrounding channel of the QWSPNb and achieve a controlled release in time and place favoring precision in the applied dose.
The calcium channel blocker VER-HCl is one of the first calcium channel antagonists introduced for therapeutic use. It presents a polar topological surface area of 64 Å2 that favors cell membrane permeability and has a high solubility in water. Its molecular size allows it to enter the central cavity through the nanobox pore for its subsequent release. One of the strategic combinations is VER-HCL and DOX-HCl whose sequential application facilitates reversing multidrug resistance from the inhibition of P-glycoprotein expression and contributes to enhance doxorubicin action (Li et al., 2015).
Other combinatorials that can be implemented to configure delivery systems are PTX and curcumin (CUR), a phenolic compound that reduces the expression of P-gp according to reported studies (Punfa etal., 2014); DT-diaphorase and methotrexate (MTX), a combination that has been reported for sequential enzyme-activated and light-triggered cancer detection and therapy (Chen et al., 2018); bio enzymes as alternative therapeutic agents, and the joint delivery of RNase A and DOX.
Sequential release of therapeutic molecular agents
To model the sequential release of the two molecular agents TM1 and TM2, the combination VER-HCl/DOX-HCl was chosen. VER-HCl was loaded into the cavity of the nanoparticle and DOX-HCl into the nanochannel. The diffusion coefficients chosen for the simulation and determined from the previously established criteria were DDOX = 0.36x10-9 m2/s and DVAR = 0.55x10-9 m2/s. The initial concentrations of the charge both in the cavity and in the nanochannel were selected according to the maximum charge capacity of the molecular agents obtained from the average dimensions of the nanoboxes. For a nanobox, the theoretical maximum capacity calculated for the DOX molecule corresponds approximately to 2x10-20 mol, which is equivalent to a concentration of 1.15 M. In the simulation, an initial concentration of 1M was assumed for both the cavity and the nanochannel. The dimensions of the nanobox were indicated above.
Figure 6 A-B shows the release profiles of the two molecular agents for a cross-section of the box under the conditions imposed by the model. As can be seen, the molecular agent in the cavity was released with a shorter half-life than that of the nanochannel. These times correspond to the case where the nanobox has not been functionalized yet with molecular agents sensitive to pH and/or temperature. The methodology and experimental development in a biological environment would allow for the evaluation of these theoretical results.
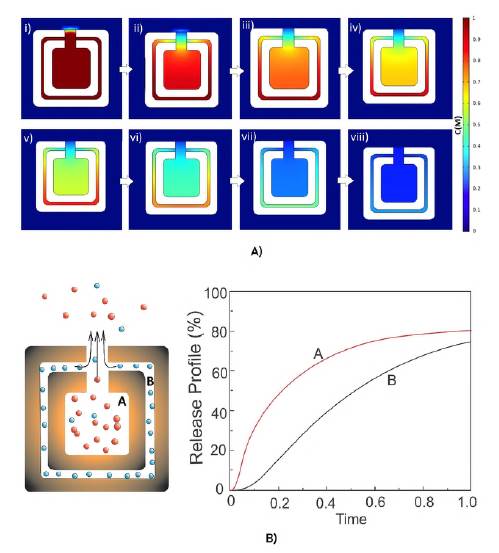
Figure 6 A) Instantaneous concentration distribution for a transverse section of the nanobox for the values: i) 0; ii) 0.08; iii) 0.16; iv) 0.25; v) 0.33; vi) 0.41; vii) 0.66; viii)1.0. It corresponds to the combinatorial AR-HCl and DOX-HCl. B) Release profile of the selected molecular agents, VAR-HCl and DOX-HCl. A corresponds to the curve from the cavity while the curve noted as B, from the nanochannel
In general, hydrophilic drugs can be loaded into the cavity and the nanochannel while hydrophobic drugs can be bound to the outer surface of the nanobox whose area is around 15,000 nm2, enough to accommodate a significant molecular cargo. On the surface, it is also possible to fix "porter polymers" to control the release of cargo through the nanopore by physical, chemical, and biological excitations, among which the following stand out: pH, temperature, light, electric fields, magnetic, among others. The abundant supply of polymers with these capabilities facilitates the design of controlled release functions for the multifunctional platform proposed here.
Polymers sensitive to pH variations contain acidic or basic groups with the ability to accept or donate protons that consequently produce changes in their structure, solubility, conformation, or surface activity (Ofridam et al., 2021). These changes can be used to open or close the exit of the nanopore. Cationic polymers subjected to pH decrease change from a hydrophobic to a positively charged hydrophilic configuration. On the other hand, anionic polymers affected by pH reductions transit from a negatively charged hydrophilic configuration to a hydrophobic one. These polymers are made up of amino groups that accept protons and make them evolve towards hydrophilic configurations when the environment pH is reduced below the pKa (Deirram et al., 2019). 2-(N,N-Diisopropylamino)ethyl methacrylate (PDPAEMA) is one of the most widely used amines for configurations sensitive to pH changes. It has a pKa acidity of 6.85 that allows for its transition towards hydrophilic with pH values below this value. For the physiological environment (e.g. blood plasma) with pH values between 7.37 and 7.43, this amino is hydrophobic. In the endosomal compartment, with pH ~6.5, and tumor environments with pH between 6.4 and 6.8, the polymer exhibits hydrophilic behavior.
Palanikumar etal. (2017) reported the use of the copolymer pyridine disulfide, 2-(diisopropylamine) ethyl methacrylate, and polyethylene glycol to control sequential delivery from a core-shell structure of hollow silica nanoparticles. Following a similar strategy but using DWSPNb with the PDPAEMA polymer as a gatekeeper at the pore exit and fixed on the surface by methods such as the one indicated in Mohammadi et al. (2017), a route for sequential and synergist treatment was drawn, as illustrated in figure 7A.
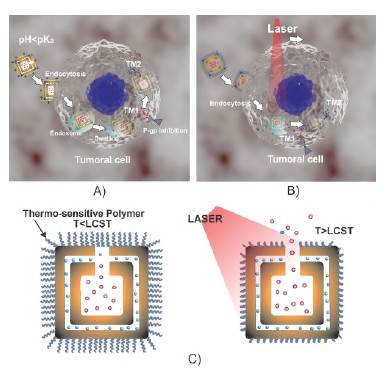
Figure 7 A) Route for sequential release of two drugs with the use of DWSPNb loaded with an MT1 blocker in the cavity and an MT2 pharmacological agent in the nanochannel. In this case, a pH-sensitive polymer is used as a gatekeeper to control the exit of molecular agents through the nanopore. B) Route for sequential release of two drugs with the use of DWSPNb loaded with an MT1 blocker in the cavity and an MT2 pharmacological agent in the nanochannel. In this case, a thermosensitive polymer is used to control the exit of molecular agents through the nanopore. C) The change in PIPAAm under the action of optical radiation which allows the controlled release of the charge is illustrated
The PDPAEMA on the surface of the DWSPNb in an environment with physiological pH presents hydrophobic behavior that allows fixing some hydrophobic drugs, for example, calcium blockers such as nimodipine (NIDIP) or VER. If the surface carries a calcium blocker, the therapeutic molecules MT1 and MT2 could be released from inside the box. In this case, there would be three molecular agents delivered sequentially. In the case of two drugs, a blocker MT1, for example, VER-HCl, and the therapeutic agent MT2, DOX-HCl, for example, the first would be loaded in the cavity and the second in the nanochannel. The PDPAEMA polymer in a tumor environment with a 6.4-6.8 pH changes towards a hydrophilic configuration producing protonation with a positive charge on the surface that favors cell internalization. Within the highly acidic endosomal environment, the polymer swells and allows the opening of the pore through which the MT1 blocker is released and the endosomal membrane is disrupted with the subsequent escape of DWSPNb. The delivery of the molecular agent MT1 blocks the P-glycoprotein responsible for the resistance to the MT2 drug. Since the pore is open, the MT2 drug is sequentially released to act efficiently thanks to the blockage of the aforementioned protein.
A second route for sequential drug delivery can be configured with the use of a thermosensitive polymer affixed to the surface of the box. This type of polymer is sensitive to temperature changes with ranges that can be programmed according to specific requirements. Those known as negative temperature-sensitive polymers are insoluble above a certain critical temperature, the lower critical solution temperature (LCST). They are soluble in water at normal temperatures (below the LCST) and their solubility decreases as temperature increases until a phase separation above the LCST value is reached.
Poly(N-isopropylacrylamide) (PIPAAm) exhibits these properties. It is classified as a smart hydrogel since it offers a wide range of applications including soft robotics, drug delivery, and tissue engineering, among others (Sanzari et al., 2020). The critical LCST value for this polymer is in the 32 to 35°C range, ideal for applications in human biological environments. At a temperature of 37oC, PIPAAm surfaces are hydrophobic favoring cell growth on them. On the contrary, at temperatures below 32°C it becomes hydrophilic. PIPAAm-coated gold nanoparticles have been widely studied due to the potential applications of this type of configuration, among which the design of nanosensors (Maji et al., 2016) and the transport and release of drugs (Esquivel, et al., 2017) have been the most researched.
DWSPNb can be targeted to the site of interest by two mechanisms: i) passive-type enhanced permeability and retention (EPR), a widely debated model that is characterized by an increased accumulation relative to normal tissue of micro and nanometric entities, such as nanoparticles or macromolecules in tumors. Presumably, this behavior is caused by the texture of abnormal vascularization within the tumor region, which promotes the translocation of nanoparticles. It has been found that this effect can be improved with the conjugation of the nanoparticles with poly (ethylene glycol). ii) The second mechanism, classified as active, takes advantage of the over-expression of tumor cell receptors that can be recognized by ligands attached to the nanoparticles allowing for vectorized accumulation at the treatment site.
As shown in figure 7B, once DWSPNb reaches the tumor cell, it is internalized by endocytosis. Inside the cell, the two molecular agents are still confined. The possibility of controlling the position of the nanoparticle in the intracellular region is being investigated. When laser light is irradiated at a wavelength belonging to the water window and the surface plasmon resonance range, the PIPAAm polymer collapses enabling the sequential release of the two therapeutic molecules (Figure 7C). The simulation of the electric field distribution and the heating of the box, as well as the control in drug delivery, act as a thermotherapeutic agent if the appropriate temperature is reached.
Conclusions
Double-walled single-pore nanoboxes (DWSPNb) synthesized by the Kirkendall effect and galvanic replacement have a structural hierarchy made up of three nanostructures: a cavity, a nanochannel, and a nanopore. According to the theoretical and computational study carried out by this research, this novel architecture allows the programming of the nanoparticle to release two molecular agents sequentially. However, taking advantage of the outer surface of the nanobox, it can also be used for the sequential transport and delivery of three molecular agents in the benefit of cancer treatment. Using polymers sensitive to variations in pH or temperature as gatekeepers in the nanopore, it is possible to configure different routes of activation and delivery of the target drugs. Besides the thermal and optical capabilities in the water window range offered by the nanoboxes, they can also become powerful nano-antennas for thermotherapy and support in diagnostic imaging tasks.
The multifunctional platform proposed here contributes from the supply of nano-technology to the investigation of potential tools to face the challenges imposed for diseases such as cancer. The option offered by these alternatives for localized and sequential delivery drastically reduces the secondary pharmacological effects, as well as the dose required to achieve the expected results. This route tracing the use of nanoparticles to transport and release drugs in a controlled manner is promising for improving the well-being of patients affected by cancer.