Introduction
As the global population is expected to increase to 8,5 billion people by 2030, 9,7 billion by 2050, and 11,2 billion by 2100 (UN DESA, 2022), the need for increased food production, particularly in developing countries, is becoming more pressing. By 2050, farmers must produce 50 % more food than in the previous decade, while also minimizing harm to the environment and reducing the excessive use of pesticides, water, and fertilizers (FAO, 2018).
Maize is one of the most important cereal crops worldwide, surpassing wheat and rice due to its high yield, affordability, ease of production and storage, versatility as feedstock or for direct consumption, and high efficiency value in industrial processing (Sprague, 1988; Vargas-Escobar et al., 2016). The global maize production was 1108 million tons in 2019 (USDA, 2020).
Plant breeding has been essential in the development of genotypes for the past century, with the inbred-hybrid concept being a significant achievement in crop breeding. Breeding methods have evolved to increase the effectiveness and efficiency of selection for multiple quantitative traits in various stages and environments (Hallauer et al., 1988).
In recent years, the development of pure lines with high levels of homozygosity in plant breeding has seen significant advancements. Traditionally, the pedigree method required a minimum of six selfing generations to achieve 99 % homozygosity. However, the doubled haploid (DH) technique has emerged as a more efficient alternative, enabling the generation of 100 % homozygous lines in just two to three generations (Geiger and Gordillo, 2009; Prasanna et al., 2019).
Initially, the DH technique relied on the in vitro anther culture protocol, but its efficiency for maize production was limited (Choudhary et al., 2014; Longin et al., 2007; Strigens et al., 2013). As a result, the technique transitioned to the in vivo method, incorporating a haploid inducer parent. This approach has gained popularity, particularly among large seed production companies, due to its ability to rapidly generate pure lines that serve as parents for hybrids (Geiger and Gordillo, 2009; Smith et al., 2008).
Developing countries are also interested in incorporating DH technology into their breeding programs, particularly in Africa, where maize hybrids that perform well under both drought stress and non-stress conditions are in high demand (Kebede et al., 2013). To produce better pure lines that are more adaptable and high-yielding, breeding programs must be more adequate and efficient. Therefore, this study aims to compare the statistical relationship among lines for hybrid combinations, highlighting the value of DH versus conventional pedigree breeding through a line by tester approach. The costs of developing lines through both methodologies will not be considered in this investigation.
Materials and methods
This research was conducted at the International Maize and Wheat Improvement Center (CIMMYT), situated in Texcoco de Mora, State of Mexico. The double haploid (DH) experiments were carried out at the Dr. Ernest W. Sprague experimental station located in Venustiano Carranza, State of Puebla.
Location
The DH laboratory, greenhouse, and fields are at the Agua Fría Experimental Station, and the nursery is in Metztitlán, State of Hidalgo, Mexico. The line by tester evaluations were conducted in four representative subtropical environments at elevations of 900 - 1800 meters above sea level during the second semester of 2016, as shown in Table 1.
Genetic material
Donor: 250 kernels from the S1 generation of ELITEWBF1/CML384 population from the CIMMYT’s maize genebank, a white flint subtropical genotype from the heterotic group B, were subjected to a double haploid process.
Inducer: The inductor 2GTHyb, a second-generation tropical adapted inducer line, was used.
Testers: Three elite white lines from the heterotic group A, CML311, CL501801, and CSL1653, known for their good general combining ability, were used to test the lines from the heterotic group B.
Check: P3055W DuPont Pioneer white hybrid maize, widely used in the subtropical region of Mexico, was used as a check.
To generate the crosses with the testers, three blocks were designed, one for each tester, with the DH line used as the female and the testers as the male.
Field test
The line by tester experiment for the three selfing generations (S2, S4, and S6) was conducted using a split-plot field design of three levels. The first level corresponded to the generation (main plot), the second level was the tester (subplot), and the last level represented the hybrids (sub-subplot). The DH hybrids were evaluated in a split-plot design of two levels, where the tester was the main plot and the hybrids were the subplot. The experimental design consisted of four rows of 4,6 m with 75 cm between rows, and 24 plants per row. The evaluation was performed in two repetitions for each of the four environments. Conventional fertilization, weed control, and native cultivation practices were employed in all environments.
Response variable
In this experiment, yield was the primary focus, but other variables were also measured, such as days to anthesis (elapsed days from planting to when 50 % of plants are giving pollen); days to silking (elapsed days from planting to when 50 % of the plants in the plot have visible stigmata); anthesis-silking interval (ASI), calculated dividing the days to anthesis by the days to silking; plant height, measured from the ground to the tip of the spike in cm; ear height, measured from he ground to the tip of the spike in cm; ear height, measured from the base of the floor to the intra node and upper ear in cm; ear position, calculated dividing the ear height by the plant height; and yield production in kilograms per hectare to 12,5 % moisture, calculated based on the grain yield per plot and moisture.
Statistical analysis methodology
Comparative analysis of adjusted testcross means in selfing and double haploid lines for grain yield
To compare the adjusted means of selfing lines and double haploid lines for grain yield, distinct procedures were necessary.
First step: To ensure the accuracy of the analysis, the data from both trails were weighted using Equation 1, which involved utilizing the standard check and nesting the lines in a grouping factor named “level”,representing the three generations and DH individually.
Equation 1. First step weight through checks
Where:
µ = General mean effect
Am = Environmental effect
Vs = Level effect (generations S2, S4, S6 and DH)(VA)sm = Level x environment interaction effect esm = Random error effect
The standard deviation for each grouping factor in the experiments was calculated using the GLM procedure in SAS 9.4. Subsequently, a new variable called “PRECISION” was created to store this information, where the value for each entry was obtained by taking the reciprocal of its corresponding standard deviation.
Second step: The response variables were weighted through the PRECISION variable in the Equation 2, using the HPMIXED procedure. To adjust the degrees of freedom, the lines were nested within the levels.
Equation 2. Second step weight through checks
Where:
µ = General mean effect
Am = Environmental effect
Gr = Grouping factor effect (level): S2, S4, S6, DH or check
(GA)rm = Grouping factor x environment interaction effect
Tj = Tester effect
(TA)jm = Tester x environment interaction effect (TG)jr = Tester x grouping factor interaction effect
(TAG)jmr = Tester x environment x grouping factor interaction effect
Ln = Line effect
(AL)nm = Environment x line interaction effect
(GL)nm = Grouping factor x line interaction effect
(GAL)rmn = Grouping factor x environment x line interaction effect
(TL)jn = Tester x line interaction effect
(TAL)jmn = Tester x environment x line interaction effect
(TLG)jnr = Tester x line x grouping factor interaction effect
(TLAG)jnmr = Tester x line x environment x grouping factor interaction effect
eijrnm = Random error effect
The random effects are:
(GB)hi/m = Grouping factor x repetition nested in the environment interaction effect
(TB)ji/m = Tester x repetition nested in the environment interaction effect
(TGB)jhi/m = Tester x grouping factor x repetition nested in the environment interaction effect
(LB)ni/m = Line x repetition nested in the environment interaction effect
(LGB)nhi/m = Line x grouping factor x repetition nested in the environment interaction effect
(TLB)jni/m = Tester x line x repetition nested in the environment interaction effect
(TLGB)jnhi/m = Tester x line x grouping factor x repetition nested in the environment interaction effect
Results and discussion
Grain yield performance among testcrosses
To assess the grain yield performance of testcrosses from selfing lines and DH lines, a model was adjusted by location and check using the check value throughout both trials to generate a standard deviation. A grouping factor effect was created to sort the data by Check, DH, and the selfing generations S2, S4, and S6 (Table 2). The PRECISION variable was then derived by dividing it by the standard deviation of each category.
Table 2 Standard deviation of grain yield from double haploid and selfing generation testcross trials from the GLM procedure
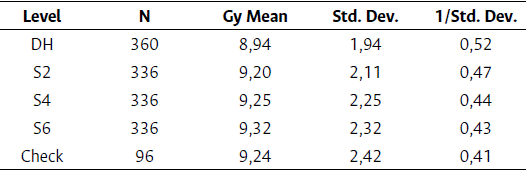
*Precision variable = 1/ Standard deviation
A new model was created using the precision variable (Equation 2), and a new matrix was generated with the S2, S4, S6 and the DH grain yield testcrosses. The WEIGHT statement in the HPMIXED procedure in the software SAS 9.4 was executed to account for the precision of the data.
The ANOVA results (Table 3) showed that the environment played a significant role in the variance of grain yield due to the range of environmental conditions in this study, even though the environments were from the same subtropical Mexican region. The model detected significant differences between the grouping factor level, indicating that at least one of the factors DH, S2, S4, and S6 is different from the others. Furthermore, there was a statistically significant difference between the testers used, and between the lines nested in the levels. Therefore, each line should be evaluated within each generation.
Table 3 ANOVA for grain yield of DH, S2, S4, and S6 selfing generations using the HPMIXED procedure
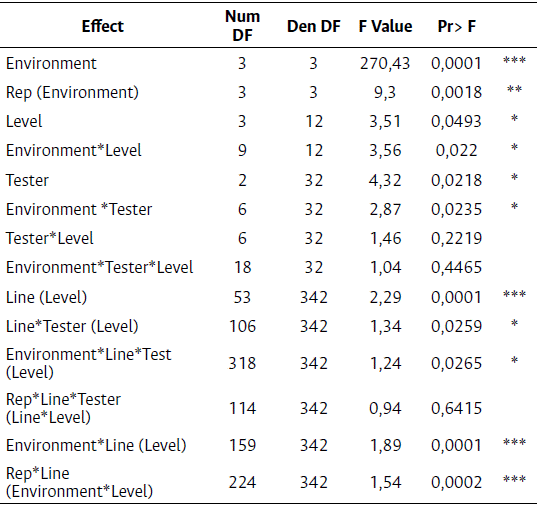
Significant Codes: 0,001 ´***´ 0,01 ´**´ 0,05 ´*´
The significant environment x level interaction suggested that the levels changed hierarchies through the different environments. In addition, there was a significant difference for the GxE interaction (Environment * Line (Level)), indicating that there was a change of grain yield hierarchies throughout all the environments. This suggests that a GxE interaction analysis should be executed for this experiment.
The HPMIXED procedure compared the levels as shown in Table 4. The results showed a statistically significant difference between DH and S4 and between DH and S6. However, there were no significant differences between selfing generations. Based on this comparison, three groups were identified: group A, made of S6 and S4; group AB, consisting of S2; and group B, comprising the DH.
Table 4 Differences in least square means for grain yield among levels
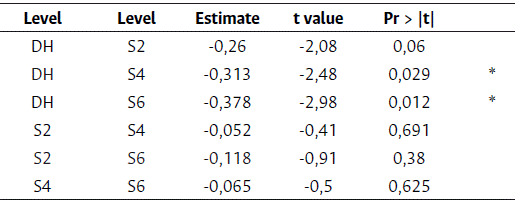
Significant codes: 0,001 ´***´ 0,01 ´**´ 0,05 ´*´
The least square means for grain yield at the different levels (Table 4) were further visualized in Figure 1, which showed the standard deviation for each level.
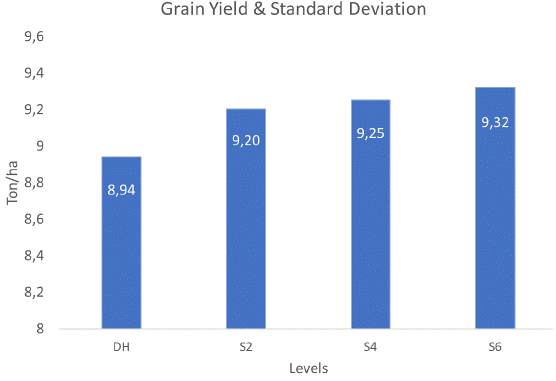
Figure 1 Adjusted means and standard deviations of grain yield in testcrosses from selfing generations and double haploids.
The evaluation of the testers was not within the scope of this investigation; the testers used were from the heterotic group A from the CIMMYT subtropical breeding program. These testers are instruments to reveal the hybridization potential of lines from the heterotic group B.
The least square weighted means for grain yield (Table 5) showed that group A had the line SL7-S4 with the highest grain yield of 9,98 Ton/ha, and group AB had two lines: SL7-S2 and SL9-S6. The top 6 lines from the list did not differ statistically from each other, and there were no DH lines among them.
Table 5 Grouping of nested lines within levels for weighted least square means (Ton/ha)
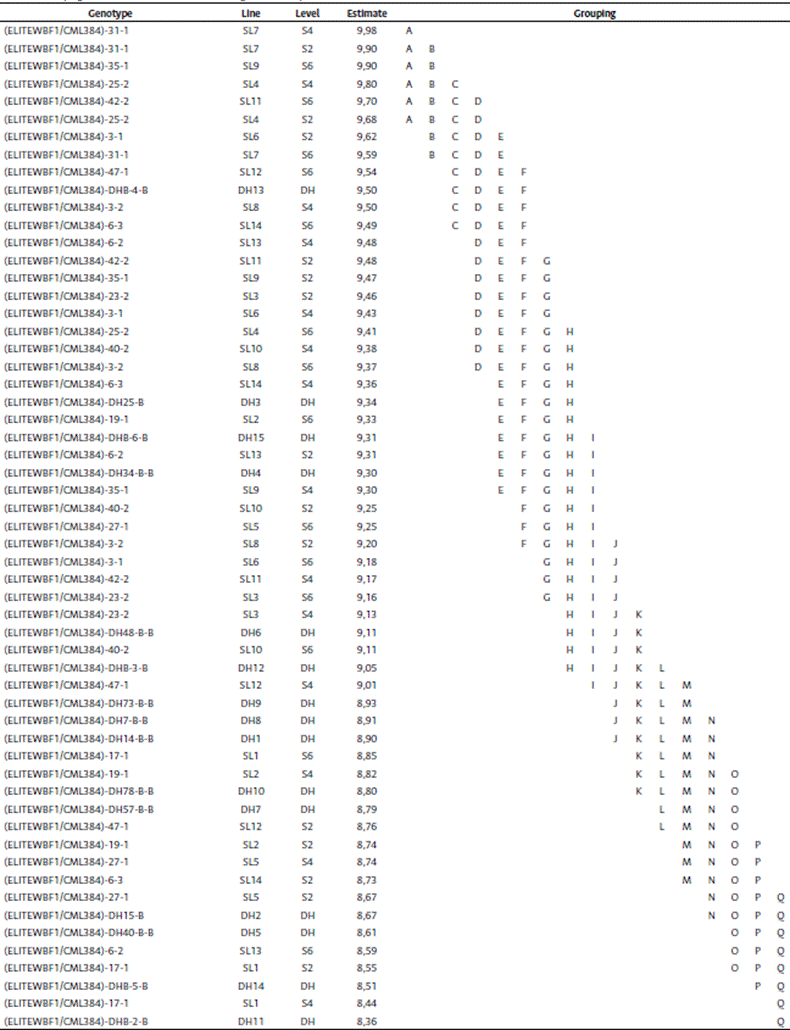
*Means with the same letter are not significantly different
*Mean yield of the testcrosses combined analysis across environments was 9,17 Ton/ha
To our knowledge, this is the first study comparing S2, S4, and S6 selfing generations with DH maize testcrosses for grain yield performance. The results suggest that, for this dataset, there is a correlation between early selfing generation testcrosses and DH testcrosses. However, as progress is made in the selfing generations, significant statistical differences can be detected for the DH lines.
In comparing our findings to previous studies mentioned in the literature review section, several similarities and differences emerged, shedding light on the performance of testcrosses from selfing lines (S2, S4, S6) and double haploid (DH) lines for grain yield in maize breeding.
One study by Bouchez and Gallais (2000) compared testcrosses of S0, S1, and S2 selfing generation plants against testcrosses of DH lines. They found that DH testcrosses exhibited an advantage in a 3-year cycle when heritability values were low. However, this advantage diminished when comparisons were made using the same effective genetic size or without off-season nurseries. In our study, we observed statistically significant differences between DH and selfing generation testcrosses, suggesting that, as progress is made in the selfing generations (S2, S4, S6), significant statistical differences with DH lines can be identified. This implies that the DH methodology may have certain advantages in terms of grain yield performance when compared to early selfing generations, but as of S4, the DH lose their comparative advantage.
Wilde et al. (2010) conducted a study where DH testcrosses were generated from three European flint-type landraces to eradicate deleterious recessive alleles. They observed that, while a significant genetic variance existed within each DH line group, the mean testcross performance of DH lines was similar to that of their parental landraces, but 22-26 % lower than present elite flint lines. In our study, we did not directly evaluate the performance of parental landraces, but we found that DH lines formed a distinct group lower from the selfing generations S4 and S6 based on the comparison of least square means for grain yield.
Statistical differences observed in this study may be attributed to the base population, specifically the S1 population composed of two elite lines belonging to the heterotic group B, while the tester belonged to the heterotic group A. As noted by Obaidi et al. (1998) citing Hallauer and Miranda (1988), the selection of the initial S0 plant for self-pollination imposes a constraint on the genetic composition, and subsequent generations mainly rely on recombination for limited additional selection. In contrast, the segregating method involves the selection of desirable traits and the deliberate removal of undesirable traits, resulting in fixation with each cycle of selection. Consequently, while genes in the DH methodology may appear randomly fixed, the segregating method exhibits a purposeful removal of undesired traits through breeding efforts.
Another possible explanation for the statistical differences observed between DH and selfing generation testcrosses is the variance analysis conducted in our study. The analysis examines both the between-group variation (variance between the levels) and the within-group variation (variance within each level). DH testcrosses, being 100 % homozygous, may exhibit smaller variances compared to the selfing generations due to their genetic stability. This reduced variance may contribute to the differences observed in grain yield performance, with the selfing generations (S4, S6) having higher mean yields compared to the DH lines.
Comparative analysis of pedigree and double haploids
Figure 2 presents a theoretical timeline outlining the evaluation process of different selfing generations using both the pedigree and DH methods for testcross evaluation. The timeline begins with an S1 population and demonstrates the parallel progression of the two approaches.
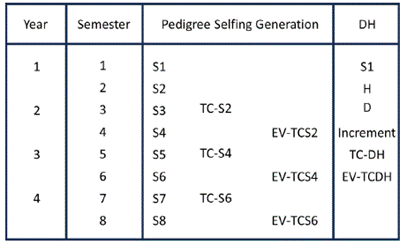
Figure 2 Timeline comparison of cycles between pedigree selfing generation and the double haploid method, starting from an S1 population. S = selfing generation, TC = test cross, EV = field evaluation, H = haploid formation, D = D0.
In the pedigree method, during the second cycle of the first year, the formation of the S2 generation takes place. Simultaneously, in the DH process, haploid induction (H) occurs. The third cycle involves the formation of the S3 generation and the cross between the S2 lines and testers (TC-S2), while the DH method involves self-pollination of the D0 in the DH nursery.
By the fourth cycle, while the S4 generation is undergoing self-pollination, the TC-S2 can be evaluated in field trials (EV-TCS2), and the DH lines (D1) continue to be incremented in DH nurseries. In the fifth cycle, the TC-DH and TC-S4 can be crossed with testers, leading to the formation of the S5 generation. Subsequently, in the sixth cycle, yield trials can be conducted (EV-TCS4 and EV-DH) while the S6 generation is being created. Elite DH lines with superior general combining ability (GCA) and outstanding characteristics can be selected at the end of the sixth cycle. Finally, by the eighth cycle, the S6 testcrosses can be chosen for GCA evaluation.
To summarize, the early generation testing (EV-TCS2) requires 4 cycles while the DH is incremented. Mid-generation testing (EV-TCS4) coincides with DH evaluation in the sixth cycle. Notably, the DH method saves one year (two cycles) compared to the pedigree-based S6 late generation testing in the eighth cycle.
Given no logistical complications and the availability of off-season nurseries or the ability to conduct two cycles per year (particularly in subtropical regions), elite DH lines can be identified from testcross evaluations within three years. Bordes et al. (2006) and Gallais and Bordes (2007) emphasize that the advantage of DHs would not exist without the possibility of executing two cycles per year due to time constraints.
Although the DH method requires additional skilled labor, training time, and resources compared to the traditional pedigree method, it allows for the rapid elimination of lines with poor agronomic performance or deleterious effects. The DH technology enables the identification of viable lines that contribute to the efficiency of plant breeding programs (Smelser, 2014).
Ongoing studies, such as those focused on accelerating and reducing the costs of DH production through techniques like embryo rescue hold the potential to enhance efficiency in colchicine treatment and the successful identification of putative haploids. Consequently, Latin American countries with plant breeding programs, where DH implementation is currently limited, may gain access to this technology.
Conclusion
The comparative evaluation of DH lines and selfing generations (S2, S4, and S6) through testcross trials for this data set highlighted notable and statistically significant differences in grain yield, favoring the segregating generations over the DH lines. While the DH technology shows promise for enhancing maize breeding programs, it should be approached as a complementary tool rather than a replacement for the well-established pedigree method.
From a timeline perspective, independent of cost considerations, the development of DH lines demonstrated its advantage by enabling the identification of elite 100 % pure lines within a three- year research period, based on efficient line-by-tester selection. Moreover, the inherent nature of DH lines guarantees the perpetuation of fixed alleles, ensuring the stability and consistency of desirable traits in subsequent generations.
This study suggests that established breeding programs with advanced elite lines may continue hybrid testing without adopting the DH mechanism. However, for new breeding programs or when introducing novel genetic materials, DH technology offers an efficient approach. Utilizing DH lines can expedite pure line development, providing a foundation for hybridization. Decisions to adopt DH technology should align with program goals, desired outcomes, and the available resources of the company. It is important to consider that DH technology can be costly to operate, which may influence its feasibility for certain breeding programs.