Introduction
Carcas schilling is an essential step to guarantee hygiene, safety, shelf life, and overall appearance of meat. This process reduces the surface temperature of carcasses, preventing growth of unwanted and harmful bacteria (Ockerman and Basu, 2004). Some preventive techniques have been used in slaughterhouses to minimize the negative impact of chilling on carcasses. A common practice consists of spraying carcasses with water during chilling. However, according to Jones and Robertson (1988), and Strydom and Buys (1995), this practice may contribute to increased microbiological contamination under unfavorable conditions.
Antimicrobial resistance can be present in several processes within the food industry and, therefore, it is considered a complex multifactorial event (Santos, 2004). Environmental spread of multiresistant bacteria is pointed out by the World Health Organization as the main responsible for increased human deaths caused by antibiotic-resistant superbugs (O’Brien, 2002). Moreover, indiscriminate use of antimicrobials (Van Boeckel et al., 2015) also contributes to bacterial selection pressure, negatively affecting prevention and treatment of bacterial infections in humans and animals (Arslan and Eyi, 2010).
Growth-promoting antimicrobials are commonly used during several stages of intensive animal production. Nevertheless, the risks to human health outweigh the benefits provided by increased productivity (Oliver et al., 2010), especially involing pathogenic bacteria. Moreover, infections caused by multiresistant bacteria increase the risk of exposure to antimicrobial drugs, particularly considering their toxicological aspects (Safdar and Maki, 2002). This situation applies mainly to the treatment and prophylaxis of animal infectious diseases (Lerma et al., 2014).
Escherichia coli (E. coli) is commonly found in human and animal gut, but it can also affect health and lead to serious infections.
E. coli contamination in animal products generally occurs through accidental spillage of fecal material onto the carcasses during slaughter, particularly during the skinning and evisceration operations (Barros et al., 2007). However, cross-contamination occurs by manipulation, inadequate hygiene of facilities and/or equipment (Borch and Arinder, 2002).
This study aimed to assess resistance to antimicrobials in Escherichia coli isolates from sheep carcasses subjected to spray-chilling with water (4 and 10 hours) during cooling.
Materials and methods
Ethical considerations
Since this study involved only the sampling and analysis of microbiological materials from sheep carcasses in slaughterhouses, approval of an Ethics Committee on Animal Use was not mandatory. However, we ensure that the sheep were slaughtered within the welfare parameters regulated by the State Inspection Program of Santa Catarina, Brazil.
Experimental Design
Antimicrobial resistance of E. coli isolates was assessed by taking samples from randomly selected carcasses in a cold room. Samples were collected by swabbing both sides of each carcass during postmortem.
In October 2015, ten carcasses sprayed with chlorinated water (1.5 ppm) for 4 consecutive hours, ten non-sprayed carcasses, and one control carcass were sampled for establishing the current health conditions at that time without the interference of those involved in the study. Therefore, these carcasses were neither included in the experiment nor manipulated by researchers, but only manipulated by the slaughterhouse employees.
In July 2016, the same sampling procedure and sample number of sprayed (n = 10) and non- sprayed carcass (n = 10) were taken. This time the carcasses were sprayed for 10 consecutive hours, and an additional carcass was added to the control group (n = 2).
Samples
The sampling technique was an adaptation of the non-destructive method by NZFSA (2008), using sterile swabs. The swabs were taken by swabbing vertically, horizontally and diagonally over a 100 cm² surface area delimited by a sterile template between the 12 and 13th ribs on the left and right carcass halves (Figure 1). In the first sampling, seven carcasses were selected and distributed, as follows: three carcasses subjected to spray- chilling using water (CWS 4 h, n = 36), three non-sprayed carcasses (NSC 4 h, n = 36) and one control carcass (COC 4 h, n= 12), totaling 84 plates of E. coli isolates. In the second sampling, eight sprayed carcasses (CWS 10 h, n = 42), three non-sprayed carcasses (NSC 10 h, n = 42) and two control carcasses (COC 10 h, n = 28) were selected, totaling 112 plates of E. coli isolates. All samples were packed in sterile Brain Heart Infusion (BHI) test tubes and immediately taken to the laboratory for processing and analysis.
Isolation and identification of Escherichia coli
The microbiological samples were homogenized in the laboratory. Then 1 mL was pipetted and incubated on six Petri dishes containing MacConkey agar medium (using the following serial dilutions: 101, 102, 103, 104, 105 and 106 CFU mL-1) (KASVI, São José dos Pinhais, PR, Brazil). In the second step, the process was repeated using seven Petri dishes (101, 102, 103, 104, 105, and 106 CFU mL-1). A total of 84 and 112 plates of E. coli isolates were obtained in the first and second samplings, respectively. Six characteristic E. coli colonies (CFU/cm²) were isolated from each plate using the dilutions indicated above. Samples were then incubated at 37°C for 18- 24 hours according to the method described by Lenahan et al. (2009).
Antimicrobial susceptibility test
Samples were diluted in a 2 mL saline solution (0.85%), and turbidity was estimated with the McFarland's scale. Subsequently, samples were incubated on Müller-Hinton agar medium (Oxoid Brasil Ltda, Pinheiros, SP, Brazil) to perform the susceptibility test using the disk diffusion method (Bauer et al., 1966).
Three groups of antimicrobials (Laborclin) were selected as standard antimicrobials. Group 1 from the first sampling (October 2015) consisted of amikacin (30 μg), amoxicillin + clavulanate (20/10 μg), ceftriaxone (30 μg), ceftazidime (30 μg), and gentamicin (10 μg). This antimicrobial combination was aimed to confirm the presence of ESBL. Group 2 included nitrofurantoin (300 μg), cefepime (30 μg), nalidixic acid (30 μg), norfloxacin (10 μg), and ciprofloxacin (5 μg). Group 3 consisted of cephalothin (30 μg), ampicillin (20 μg), meropenem (10 μg), sulfonamide (300 μg), and tetracycline (30 μg). The same antimicrobials used in the susceptibility test for the first sampling were used in the second sampling (July 2016), but chloramphenicol (30 μg) and trimethoprim (25 μg) were added to the second group. E. coli strain ATCC 25922 was used as a control. Reading and interpretation of the results were performed according to the Clinical and Laboratory Standards Institute (CLSI, 2015). Multiresistant isolates demonstrated resistance to three or more classes of antimicrobials (Magiorako et al., 2012). Determination of multiple antibiotic resistance (MAR) index was conducted according to the methodology described by Krumperman (1983).
Statistical analysis
A bivariate analysis using Pearson’s correlation with p<0.05 as significant was performed to test for heterogeneity or linear trend between treatments, considering the prevalence of bacterial resistance in each class of antimicrobials and their respective multi- drug resistance. A 95% confidence interval was considered for all tests. Additional calculations were performed using the Epi Info 7 software.
Results
The slaughtering process involves several critical points that could influence the results obtained in this study in terms of microbial contamination. Carcasses sprayed for 4 hours (CWS 4 h) showed resistance to cephalothin (94%, 34/36), nalidixic acid (19%, 7/36), and sulfonamide (25%, 9/36), as shown in Table 1.
Isolates from non-sprayed carcasses (NSC 4 h) had the highest percentage of resistance to cephalothin (97%, 35/36) and the lowest percentage of resistance to ampicillin + sulbactam (17%, 6/36), amoxicillin + clavulanate (11%, 4/36), nitrofurantoin (19%, 7/36), and nalidixic acid (14%, 5/36). The following percentages of resistance were obtained for isolates from the control group (COC 4 h): cephalothin (83%, 10/12), ampicillin + sulbactam (42%, 5/12), amoxicillin + clavulanic acid (33%, 4/12). Resistance to nalidixic acid (14%, 5/36) of isolates from non-sprayed carcasses was higher than 10%, which warns public health risk, especially regarding the presence of residues in meat.
All 17 antimicrobials tested in E. coli isolates from carcasses sprayed for 10 h (10 h CWS) during the second sampling presented antimicrobial resistance higher than 10% (Table 1). Antimicrobials amikacin (7%, 3/42), gentamicin (4%, 2/42) ciprofloxacin (0%), norfloxacin (0%), and sulfonamide (7%, 3/42) showed low resistance in carcasses of the treatment NSC 10 h. The highest percentage of resistance was observed to cephalothin (83%, 35/42), followed by ceftriaxone (31%, 13/42), ampicillin + sulbactam (60%, 25/42), amoxicillin + clavulanate (83%, 35/42), nitrofurantoin (60%, 25/42), nalidixic acid (31%, 13/42). Four out of 17 antimicrobials studied belong to the class of β-lactams. The control group (COC 10 h) showed higher percentage of resistance to cephalothin (18%, 5/28), ceftazidime (18%, 5/28), ceftriaxone (14%, 4/28), meropenem (14%, 4/28), nitrofurantoin (18%, 5/28), nalidixic acid (11%, 3/28), tetracycline (11%, 3/28) and trimethoprim (14%, 4/28). Resistance to meropenem was observed in spray-chilled carcasses (19%, 8/42) and the control group (14%, 4/28).
In E. coli isolates of the second sampling, the highest resistance to antimicrobial classes were detected for nitrofurans (60, 60 and 17%), sulfonamides (7, 7 and 25%) and β-lactams (24, 18 and 16%) in sprayed, non-sprayed carcasses, and control group, respectively. When comparing the number of resistant antimicrobial classes between E. coli isolates of the first and second samplings (Figure 2), the most significant values were observed for carcasses sprayed and non-sprayed for 10 hours.
Table 1 Percentage (%) and absolute frequency (AF) of resistant E. coli isolates from sheep carcasses subjected to spray-chilling with water (CWS) and non-sprayed carcasses (NSC) at different sampling moments (2015 and 2016).
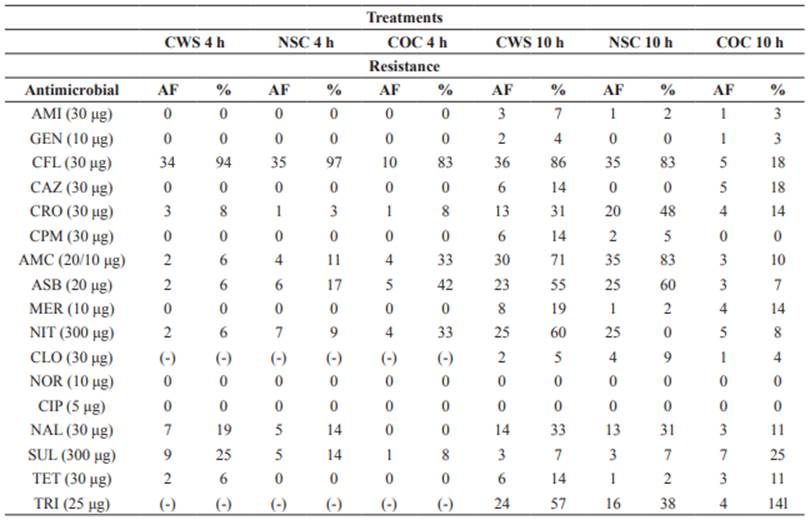
CWS 4 and 10 h = carcasses subjected to spray-chilling using water for 4 and 10 h; NSC 4 and 10 h = non-sprayed carcasses; COC 4 and 10 h = control carcass; AF = absolute frequency; (-) = antimicrobial not tested; AMI = amikacin; GEN = gentamicin; CFL = cephalotin; CAZ = ceftazidime; CRO = ceftriaxone; CPM = cefepime; AMC = amoxicillin + clavulanate; ASB = amoxicillin + subactam; MER = meropenem; NIT = nitrofurantoin; CLO = chloramphenicol; NOR = norfloxacin; CIP = ciprofloxacin; NAL = nalidixic acid; SUL = sulfonamide; TET = tetracycline; TRI = trimethoprim.
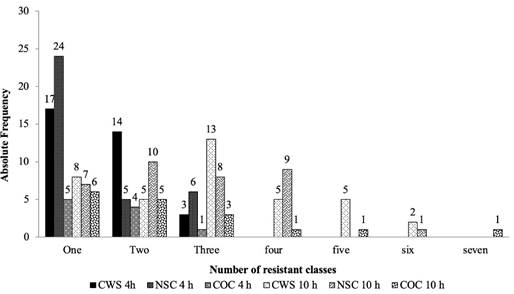
Figure 2 Absolute frequency of antimicrobial-resistant E. coli isolates per class tested in the first (October 2015) and second sampling (July 2016).
The multiple antibiotic resistance (MAR) indexes of spray-chilled, non-sprayed, and control carcasses were 0.3, 0.1, and 0.1, respectively. These values reinforce concerns regarding cross-contamination during the manipulation of animal products. Relative to samplings periods, the values were statistically significant (Table 2) (p<0.05) only when comparing NSC 4 h x NSC 10 h (p = 0.006) for one class, CWS 4 h x CWS 10 h (p = 0.0577) for two classes and NSC 4 h x NSC 10 h (p = 0.0211) for four classes, in which spray- chilled carcasses were more multiresistant. No significant results were found for comparisons within treatments.
Table 2 Statistical significance of antimicrobial-resistant classes tested in E. coli isolates from sheep carcasses subjected to spray-chilling with water in the postmortem period.
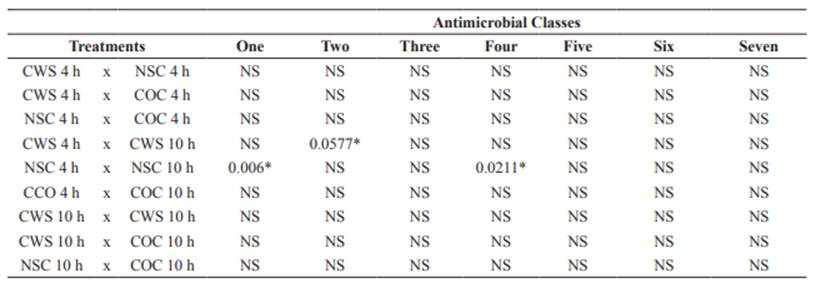
CWS = Carcasses subjected to spray-chilling using water for 4 and 10 h; NSC = Non-sprayed carcasses; COC 4 and 10 h = Control carcass, NS = not significant, *Significance declared at p<0.05.
Discussion
We found high levels of antimicrobial resistance in carcasses subjected to long spray- chilling with water. Regardless of treatment, it is noteworthy that antimicrobial resistance was significant for most antimicrobials, mainly for β-lactams. A previous study showed that antimicrobial resistance might involve three mechanisms:decreasedaccumulationofthedrugs by the cell, hydrolysis of antimicrobials, and/or reductions in drug affinity due to alterations in binding proteins (Miró et al., 1994). In addition to the ability of bacteria to produce β-lactamases, no extended-spectrum β-lactamases (ESBL) were found among E. coli isolates in this study.
In the first sampling, carcasses sprayed for 4 hours were resistant to β-lactams, quinolones, and sulfonamides, while non-sprayed carcasses showed resistance to β-lactams, quinolones, and nitrofurans. According to Phillips et al. (2004), antimicrobials can select resistant mutant bacterial strains through resistance transferred from other bacteria. The resistance to quinolone in gram-negative bacilli is mainly attributed to gene mutations that encode quinolone targets and alterations in external membrane proteins or efflux pumps (Strahilevitz et al., 2009; Lindgren et al., 2003). Overall, these results corroborate previous studies conducted by Sáenz et al. (2001) and Dontorou et al. (2003), which indicate resistance to the main classes of antimicrobials in E. coli isolates from animal products.
Studies by Rahamathulla et al. (2016) showed that resistance to meropenem is an increasing public health problem because this antibiotic is one of the last resources for the treatment of infections in hospitals. Rasmussen et al. (1996) reported that this is associated with genes encoding carbapenemases on plasmids or transposons in carbapenem-resistant bacteria. In E. coli, these genes are responsible for resistance to carbapenems due to the presence of an outer membrane protein deficiency and expression of plasmid-mediated class C beta- lactamase gene (Stapleton et al., 1999). The E. coli isolates from the control carcasses showed resistance to meropenem, possibly due to cross- contamination of bacteria with the resistance gene for this carbapenem during slaughter.
The present study indicated that E. coli isolates from carcasses subjected to spray-chilling with water had greater antimicrobial resistance, especially in carcasses sprayed for 10 h, even with the recommended chlorine level. Longer spray- chilling time facilitated the dispersion of bacteria on carcasses, which may increase contamination of carcass surfaces. Moreover, spray-chilling increased both colonization of bacteria and their survival under cooling conditions.