1. INTRODUCTION
Public transportation, indispensable in the modern world, provides the opportunity to meet the increasing demand for urban mobility while supporting socioeconomic development. To achieve environmental benefits in public transportation systems, the concept of sustainable transportation is essential. Transportation in urban areas, including cars and buses, contributes largely to energy consumption and greenhouse gas emissions. A comprehensive understanding of zero-emission approaches is crucial to assess current knowledge on this topic. Existing literature suggests that promoting public transportation, biking, walking, and the use of electric and low-emission vehicles are effective methods for reducing energy consumption and greenhouse gas emissions in urban areas. However, there is a need to identify gaps in existing zero-emission approaches for potential future studies. Mobility in cities is about % of the world's energy consumption; it also corresponds to 70% of man-made greenhouse gas emissions (Difiglio et al., 2020). The total energy demand in transportation worldwide was 90% met from oil in 2020. In addition, the transportation sector accounts for 60% of the world's total oil demand. Transportation is the final energy consumption sector with the highest dependency on fossil fuels. It has been stated that 37% of total CO2 emissions in final energy consumption on a global basis originated from the transportation sector in 2020 (Birol, 2021). The public transportation sector, which is based on a zero-emission concept, has significant potential in terms of being carried out from a single source, economies of scale (Topal & Ates 2021). Zero-emission concepts in public transport are seen as an important step in improving local air quality by reducing greenhouse gas emissions. Commitments are widely expressed to fully electrify the vehicle fleets on highways from 2030 to 2050. (IEA, 2021). According to current data published by the International Energy Agency, greenhouse gas emissions due to the amount of fuel used by the transportation sector worldwide, increased by 7.01% compared to the previous year and urban public transportation systems account for 40% of CO2 emissions from all road transport (Topal, 2021).
In this study, an alternative approach for Rubber-Wheeled Transportation Systems in public transportation is used. A new model based on indexed to passenger ticket income specified for the Istanbul Metrobus System. It has been proposed based on real operating parameters and current costs to relevant audiences. In this sense, the ticket income-indexed with Payback Period Method is implemented for the fuel cell hybrid-electric buses. Also, it has been put forward for the first time on the public transportation sector, where the use of battery-electric buses is becoming increasingly common in Türkiye.
The remainder of the paper is structured as follows: Section 2 shows zero emission concept approaches in public transportation, information on recent improvements, and literature studies on this topic. Section 3 focuses on the general methodology of the study and information about alternative powertrain for bus concepts. Section 4 puts forward the main concept, describing the methodology and data used in the study. Section 5 presents the results and discussion of the analysis. Finally, Section 6 concludes the study and offers suggestions for future research.
2. ZERO-EMISSION CONCEPT APPROACHES IN PUBLIC TRANSPORTATION
China stands out in the use of alternative fuel buses for public transportation concepts around the world. Thus, electric buses accounted for a 27% market share by the end of 2020 in China. China's fleet has approximately 700,000 electric buses, which corresponded to more than 90% of the electric buses worldwide in 2022 (Rodrigues & Seixas, 2022). It has been stated that in line with London's public transportation strategy, a carbon-free city concept model is aimed until 2050, and similar approaches are followed by Tokyo, New York, and Los Angeles (Sen & Miller, 2022). In Paris, the buses used in public transportation will be made electric and biofuel by 2025; it is stated that the use of internal combustion engine buses will not be allowed in 2030. Acting under the global trend, Russia has declared that as of 2021, only electric buses will be purchased (Kudryavtseva et al., 2021). Denmark has 100% zero-emission targets for newly purchased public transport buses from 2025; New Zealand will only allow the purchase of buses suitable for public transport that comply with the zero-emission concept. In the Netherlands, only battery-electric buses or fuel cell hybrid-electric buses will be preferred in all newly purchased buses for public transportation with the aim of reducing greenhouse gas emissions to zero by 2025. In addition, the Netherlands ranks first in Europe in the use of electric buses; in this respect, it is stated that 81% zero-emissions are provided in the newly purchased city buses (Grütter & Grütter Consulting, 2014). In line with carbon neutral targets, Austria aims to reach 2032 and Cape Verde chose 2035 as reference year for the transition to 100% zero-emissions. Chile, in South America, states that as of 2021, alike Colombia, will switch to a 100% electric or zero-emission approach for new purchases or buses in use by 2035. It is stated that this approach will be the basis for BRT systems, which is especially common in Colombia (Díez et al., 2021). The US state of California states that the process to be carried out within the scope of zero-emission of new buses to be purchased in public transportation will be implemented gradually, aimed at reaching 100% in 2029 (Collantes & Sperling, 2008). While the share of zero-emission buses in the European Union was 4% in 2019, it increased to 6% in 2020 (European Automobile Manufacturers' Association, 2021). EU Member States have set minimum targets in line with the zero-emission approach for bus purchases in public transport systems in 2019. It is stated that these targets vary between 24% and 45% in 2025 and between 33% and 65% in 2030, depending on the population and gross domestic product of the countries. Also, some alternative fuel-powered propulsion systems such as plug-in hybrids, CNG, and LPG are also described as clean. Nonetheless, according to the relevant directive, it is stated that the target scales should consist of at least 50% of battery-electric or fuel cell hybrid-electric buses (Moreno & García-Álvarez, 2018).
Many countries around the world have made progress within the framework of the zero-emission public transportation approach. However, no country has so far introduced a binding regulation on new bus purchases for public transport systems. The basic perspective is considered a commitment to completely decarbonize bus fleets in coming years. In Table 1, within the framework of the agreements made based on countries and the published delegations, the processes for suspending the use of internal combustion engine vehicles, and the target is for new purchases/sales of 100% zero-emission public transportation buses and other medium/heavy-duty vehicles. In this context, technical limitations on the current use of battery-electric buses, fuel cell hybrid-electric buses are considered a suitable alternative in line with these targets for the medium and long term.
Electric and hydrogen-based propulsion systems come to the fore in innovative solutions aimed at reducing CO2 emissions for urban public transportation system (Berger et al., 2015). Alternative fuels, which are characterized by low CO2 emissions, have been determined as the main target of the sustainable transportation strategy that started all over the world (Chang et al., 2019). Fuel cell hybrid-electric buses together with the hydrogen concept produced from renewable resources which are the most important alternatives for the zero-emission approach on the axis of sustainable public transportation. Compared to the most widely used diesel buses in the current situation, it has been demonstrated that it has the potential to provide approximately 93% reduction in CO2 emissions. However, it is seen that they have approximately 4-5 times higher procurement costs compared to the diesel buses in public transportation (Deliali et al., 2021). It is known that there are economic and technical obstacles in the widespread use of hydrogen fuel cell hybrid-electric buses. Similarly, performance data and environmental impacts are also discussed. It is evaluated that innovative approaches based on the life cycle cost analysis should be put forward in future studies and analyses based on the effect of main parameters such as vehicle life cycle and hydrogen production models should be emphasized.
According to studies carried out by public transportation operators/authorities and bus manufacturers, especially local governments, in line with the targets set, it is stated that electrification of used public transportation bus fleets is possible by choosing suitable concept buses for new purchases, or by converting existing conventional vehicles (Gabsalikhova et al., 2018; Melo et al., 2014). In this context, the zero-emission approach that electric and fuel cell hybrid-electric buses will provide advantages in many ways such as reduction of noise levels and vibration due to the use of electric motors in propulsion systems, thus increasing travel comfort and improving driving dynamics (Laib et al., 2019; Correa et al., 2017).
There is a tendency towards battery-electric buses, mostly among public transportation operators and authorities. Fuel cell hybrid-electric buses have two main advantages over battery-electric buses. First, the fuel (hydrogen) refuelling time is much faster, which is similar to the refuelling times of the diesel buses that are widely used in current public transportation companies. Second, considering the high hydrogen density, longer-range values can be reached with a single replenishment. The energy consumption and emissions of diesel, battery, and fuel cell hybrid-electric buses depend on the size and year of use of the bus, range travelled, road condition and speed, etc.. Fuel consumption values of a 12 m. diesel bus, mostly used in public transportation, vary from 28 lt/100 km to 65 lt/100 km (Ally & Pryor, 2016). In the bus fleet serving on a route integrated with the metro line in Australia, the average fuel consumption has been measured to be approximately 40-45 lt/100 km (Mulley et al., 2020). While this value is about 40-55 lt/100 km in the Istanbul public transportation system, it goes up to 60 lt/100 km in the istanbul Metrobus System (IETT, 2021; Topal & Nakir, 2018), Chang has compared the CO2 emission levels of buses serving Tainan, Taiwan. Emission levels are 63.14 g CO2-e/km for CNG buses, 54.6 g CO2-e/km for diesel buses, 47.4 g CO2-e/km for LPG buses, 37.82 g CO2 -e/km for battery-electric buses and 29.17 g CO2-e/km for fuel cell hybrid-electric buses (Chang et al., 2019). Similarly, the emission levels of conventional propulsion (diesel) buses in the United Kingdom and the emission levels of battery and fuel cell hybrid-electric buses were compared by Logan. Considering various electricity and hydrogen production processes in the study, the emission value at full capacity of an internal combustion engine (diesel) bus is 16.3 g CO2 -e /km, 5.3 g CO2 -e/km for battery-electric bus and 12.9 g CO2 -e for fuel cell hybrid-electric bus (Logan et al., 2020).
The performance tests were conducted by Solaris and VDL. It has been reported that the energy consumption of battery-electric buses varies depending on outdoor temperature, cooling & heating functions, driving profiles, and other road-based operating parameters. According to the data prepared by the OEMs, the electric bus with a battery capacity of 300 kWh, can reach a range of 375 km, based on appropriate conditions. However, it is stated that this value decreases to 130 km in harsh weather and road conditions. The results obtained also vary significantly as to the total emission value (depending on the electrical energy consumed for the same distance travelled). Especially for air conditioning systems, based on conventional systems, it can reduce electrical energy consumption in hybrid structures. It is indicated that due to the accompanying fossil fuel consumption, more emission production will occur in total (Al-Saadi et al., 2022).
Regardless of the production process of the fuel/energy used for the public transportation sector, according to the tank-to-wheel emission measurement index, the emissions caused by fuel cell hybrid-electric buses are negligible. For example, with the Hyundai Nexo (small class, fuel cell electric vehicle), 1 kg of hydrogen consumption allows to travel up to 100 km. Fuel (hydrogen) consumption in buses is reported to be up to 9 kg per 100 km. Accordingly, fuel cell hybrid-electric buses can reach a range of 300 to 450 km without refuelling. While this value corresponds to similar performance results with diesel buses of the same quality, it reveals a significant advantage over battery-electric buses (Pawele et al., 2020).
A case study was conducted in Australia based on the total cost of diesel, CNG, hybrid and hydrogen fuel cell buses. It has been reported that the obtained range value varies depending on parameters such as total operating time, stop/start times/number, idle running percentage, daily operating frequency and average speed values. Therefore, it states that these parameters are closely related to the energy consumption of buses (Mulley et al., 2020).
It is mentioned that the energy consumption of battery-electric buses will vary according to factors related to road conditions (Friedlingstein et al., 2020). Other factors that can affect energy consumption include tires, air conditioning use, vehicle length, weight and drive/transmission systems. The study revealed that a 15% reduction in the rolling resistance of bus tires corresponds to a 3% reduction in energy consumption, and that rolling resistance can be reduced by up to 30% with the developed tire design technologies (Friedlingstein et al., 2020). Similarly, Ritari has investigated the relationship between determining the appropriate and optimum gear ratio of battery-electric buses and their energy consumption and costs. He shared the results that, compared to the fixed gear ratio, a two-ratio gearbox consumes about 3% less energy, and a continuously variable gear consumes 4% more energy (Ritari et al., 2020).
Numerous studies in recent years have shown that fuel cell hybrid-electric buses, using renewable energy sources, are the optimum solution in public transportation systems (Guerra et al., 2018). It is reported that public transportation fleets built from fuel cell electric buses are becoming increasingly common in Europe. In addition regarding bus purchases, it is suggested that additional infrastructure costs are required for hydrogen production and supply (Navas-Anguita et al., 2020).
Santarelli conducted a study on fuel cell buses for the first time in 2003. In that study, fuel cell hybrid-electric buses were evaluated in terms of emissions and economy. However, the costs used are far beyond current conditions and are based on the relevant period (Santarelli et al., 2006). A study was also conducted by Doyle, which shows the environmental and social benefits of alternative powertrain bus concepts in reducing concerns about emission emissions and energy security issues caused by local governments. He investigated the suitability of fuel cell hybrid-electric buses and battery-electric buses from the point of view of public transport operators, based on different case studies performed in this context (Ajanovic et al., 2021).
An environmental assessment of the life cycle of fuel cell hybrid-electric school buses in the USA was conducted by Lee et al. It is stated that results can vary significantly depending on operating cycles of the buses, road conditions, hydrogen production methods, and regional power grids (Lee et al., 2011). Correa et al. compared the performance of different types of urban public transportation buses. In this regard, analyses have been carried out considering different production options of green hydrogen for fuel cell hybrid-electric buses (Correa et al., 2019). Based on rather optimistic assumptions in 2010, Bonilla and Merino assessed the feasibility of a fuel cell hybrid-electric bus incorporating carbon credits and subsidies (Bonilla et al., 2010). Cockroft and Owen conducted a cost-benefit analysis comparing diesel and CNG buses and fuel cell hybrid-electric buses in the bus fleet in the city of Perth, in line with the assumptions that appropriate economies of scale and necessary fuel infrastructure conditions are provided (Cockroft & Owen, 2007). A comprehensive review of fuel cell applications in the automotive industry was conducted by Olabi et al. In the study, the difficulties related to the use of fuel cells in the transportation sector are included based on different transportation modes (Olabi et al., 2021).
Lian et al. present a multi-objective real-time energy management strategy for fuel cell/battery plug-in hybrid electric buses based on actual operating condition data of Dalian city buses. The strategy relies on predicted motor power and a real-time reference State of Charge (SOC) to design a model predictive control strategy for solving multi-objective cost functions to achieve optimal power allocation (Lian et al., 2023).
The study on hydrogen fuel cell vehicles used in mass transportation systems in Qatar proposes several regional policies, including gradual reduction of subsidies for gasoline and other fossil fuels, incentives for fuel cell vehicles, diversification of alternative fuel-powered mass transportation, and additional restrictions on vehicle exhaust emissions. These policies are recommended to promote the adoption and use of hydrogen fuel cell vehicles in the region, as reported by Mendez et al (Mendez et al., 2023).
Broatch et al. developed a novel global model to evaluate different strategies for the integrated thermal management system of a fuel cell electric bus (FCEB) in Valencia, Spain, under winter driving conditions. Strategies included using fuel cell heat for cabin heating (up to 7% savings), waste heat to preheat batteries (4% savings under high-power demand), and a hybrid solution that uses residual heat for both cabin and battery heating (10% savings) (Broatch et al., 2023).
3. GENERAL METHODOLOGY OF THE MODEL AND MAIN PARAMETERS
The proposed model uses the Payback Period Method, which intends to compare three main bus concepts. The study focuses on the main bus concepts derived from the Fébus Project in France, the Metrobus System, and the Elbus Project in Turkey. The operational and purchasing costs' data were used as the basis for such analysis. Moreover, given the involvement of two different countries, the model incorporates a special adjustment to account for the differences in labor costs related to the maintenance and repair costs of bus concepts.
In this study, a new approach is proposed for the transition of the Istanbul Metrobus System towards a sustainable, zero-emission public transportation concept. The approach is based on Life-Cycle Cost Analysis (LCCA) with an index linked to passenger ticket revenue, a novel method for evaluating sustainability that utilize fuel cell hybrid-electric buses. The flowchart of the proposed model is presented in Figure 1.
It is clear from Figure 1 that the proposed model consists of five steps. These calculation steps are described below.
Step 1 (Performance Results): The first step of the model is putting forward the vehicle performance results under actual field conditions. In this paper, the result of the performance test for the various bus types considered are obtained in Fébus Project, Metrobus System and Elbus Project. Details of the bus performance results are explained in Section 3.
Step 2 (Creation of Data Source): Data source is created in two main sections; the first is the purchasing costs of bus concepts, and the necessary infrastructure installation costs. The seconds one is operational costs. The analysis based on current data belongs to Fébus Project, Metrobus System, and Elbus Project. The details and analysis results of the model are shown in the following sections.
Step 3 (LCCA Analysis): Life-Cycle Cost Analysis are performed in the next stage of the proposed model. The proposed method takes into account the bus purchases, and the operational investment costs depend on the variables of transportation operators. The cost components considered in this model are given in the onward sections.
Step 4 (Financial Model): Life-Cycle Cost Analysis and the Payback Period (PB) are then performed to compare the various bus concepts.
Step 5 (Evaluation of Economic Analysis and Comparison of Bus Concepts): In the final section, diesel bus, battery-electric bus, and fuel cell hybrid-electric bus are compared based on the economic analysis performed. In this section, the bus purchasing costs, operating costs, and other investment costs for infrastructure are considered as central variables, which are dependent upon transportation programs.
Also, the aforementioned financial analysis anticipated the development. Using the proposed calculations, it is possible to analyze payback and amortization periods for fuel cell hybrid-electric buses as compared to diesel and battery-electric buses. The proposed model also facilitates a precise comparison among popular concepts according to most important public transportation agencies in Turkey. Further details and calculations regarding the model are described in the following section.
FUEL CELL HYBRID-ELECTRIC BUS CONCEPT AND POTENTIAL INTEGRATION IN ISTANBUL METROBUS SYSTEM
Generally, there are three basic system configurations in propulsion systems based on hydrogen technology. These are the conventional systems driven by the use of hydrogen directly by the internal combustion engine. The second system, is driven only by the fuel cell, and the last one is the fuel cell hybrid-electric propulsion system. The first one is related to internal combustion systems. In this case, energy conversion is provided by burning hydrogen directly. In propulsion systems where only fuel cells models are used, energy is provided directly to an electric motor and regenerative energy recovery is not possible during braking. Hence, the most efficient propulsion system solution using hydrogen technology is the fuel cell hybrid-electric propulsion system, which is also the basis of this study. Thus, an integrated solution with energy storage systems can be offered in the form of a fuel cell integrated battery, ultracapacitors, or a combination of both. It corresponds to fuel cell hybrid-electric bus concepts, and these systems are also called range extenders.
The low energy density of the batteries and the need for charging infrastructure for electric buses in sustainable public transportation also increase the initial costs (Kim et al., 2021). The range values that can be achieved with battery-electric buses may adversely affect public transportation companies due to the limitations in existing systems. The basic theory that needs to be put forward is the charging system, plus the battery capacity optimization approach based on operating conditions. Electric bus concepts with overnight charging with high battery capacity or the fast charging concept with low battery capacity have various comparative advantages and disadvantages. This highlights the fact that fuel cell hybrid-electric buses offer an optimum solution. In terms of uninterrupted and sustainable transportation, which is the fundamental element of public transportation, several uncertainties persist as to battery-electric buses with high/low battery capacity and fast/slow charging systems, which has to do with both, authorities and operators (Topal, 2017).
The use of electricity or hydrogen produced from renewable energy sources in battery or fuel cell hybrid-electric buses brings CO2 emissions to a negligible level. Main hydrogen production processes that can be used especially for fuel cell hybrid-electric buses are steam methane formation and solar and wind-assisted electrolysis (Saltzstein et al., 2020). According to the Clean Energy Finance Corporation, about 95% of the world's hydrogen production comes from the use of natural gas or other hydrocarbons.
Fuel cell hybrid-electric buses use hydrogen to power the electric motor, using fuel cell technology. Buses based on this concept, which emerged with the fuel cell system added to the battery-electric bus system architecture, have been used commercially since 2014. However, due to the widespread lack of hydrogen supply chains, and refuelling stations, and the high initial cost of purchasing (compared to battery-electric buses and the other conventional buses), it has not reached a sufficient level of prevalence (Wu et al., 2021).
Approximately 11,000 fuel cell vehicles were sold in 2020. It is stated that approximately 40,000 units have been reached in the global vehicle market, and 15% of these are fuel cell hybrid-electric buses that are the subject of the study (Baldino et al., 2021). In this sense, it is stated that the hydrogen refueling station where the hydrogen to be used for fuel cell hybrid-electric vehicles is refueled has increased by 15% worldwide, reaching a total of 500 units by 2020. Considering their distribution by country, Japan is the leader with 113 stations, 81 stations are located in Germany, and 64 stations are located in the USA, respectively. In this context, it is worth noting that the number of hydrogen refueling stations in China, 61 units, almost trebbled in 2019 (Dincer et al., 2021).
The use of fuel cell hybrid-electric buses has increased in recent years, supported by various incentive mechanisms and the implementation of policies worldwide. Nevertheless, the adoption rates of these buses remain relatively low compared to battery-electric buses. The most important advantage of fuel cell hybrid-electric vehicles is the fueling times of 3 to 5 minutes, and the range they offer, depending on the type of vehicle and the desired range, thanks to their high energy density. In this respect, it provides a similar operating performance to that of conventional bus concepts. This feature makes fuel cell hybrid-electric buses an important alternative to the currently used battery-electric buses, especially for public transportation systems operating in harsh conditions. In this context, fuel cell hybrid-electric buses represent an important potential for the public transportation sector, offering a sustainable option with 100% zero emissions (Difiglio et al., 2020).
Reviewing public transport service providers around the world, the fuel cell hybrid-electric buses currently used are mostly state-sponsored or financed by EU projects. Some cost data used in the analyses presented in this study are based on JIVE1&2 and CHIC projects (Kozlov et al., 2021).
Well-to-wheel efficiency and emission factors were considered in the environmental assessments of fuel cell hybrid-electric buses. Another environmental assessment criterion is the noise level from the propulsion system. Performance evaluations within the scope of this study focus mainly on the range value achievable with fuel cell hybrid-electric buses. Evaluations consider factors such as flexibility of public transportation system routes, fuel/energy supply time, necessary infrastructure requirements, and engine's performance (torque/speed). Ultimately, a comprehensive approach based on the financial Life-cycle Cost Analysis has been introduced.
In this paper, a fuel cell hybrid-electric bus for the Istanbul Metrobus Line is presented. Accordingly, fuel cell hybrid-electric buses, battery-electric buses, and conventional buses (diesel buses currently in operation) were compared based on environmental, performance and Life-cycle Cost Analysis criteria. In comparisons based on different propulsion systems used in public transport systems, fuel cell hybrid-electric buses have the potential to actually deliver 100% zero-emissions from well to wheel. The emission value changes depending on the method of obtaining the energy used in powertrain systems. Fuel cell hybrid-electric buses, using hydrogen produced with renewable energy support, offer a significant environmental advantage as compared to battery-electric buses using electricity generated by conventional methods, aligned with the carbon-neutral goals of public transportation authorities/operators.
On the other hand, another environmental noise level factor is the noise emitted by the powertrain bus system; propulsion systems with electric motors offer advantages over conventional propulsion systems. Thus, the noise of the fuel cell hybrid-electric bus is 3 times lower than that of the internal combustion engine (diesel) bus. It is also reported from the Fébus Project, where fuel cell hybrid-electric buses are used, that a comfortable and trouble-free journey experience is provided due to the low noise level of the buses, which translates into a passenger satisfaction rate of 82% (Kempf, 2022). Also, Figure 2 below shows the noise level measurements in public transport bus systems with different propulsion systems (Undertaking, 2012).
The range performance of fuel cell hybrid-electric buses is similar to that of conventional powertrains (diesel and CNG buses). On the other hand, trolleybuses that can reach long-range values thanks to the catenary line, unlike battery-electric buses (with fast or slow charging concept), are similarly disadvantaged as they can provide service along their limited routes (along the catenary line they are connected to). Different from other electric bus concepts, fuel cell hybrid-electric buses offer sustainable and uninterrupted operation in different conditions with daily operation routines. It can continue its operation requiring long-range values with no hydrogen refueling. Furthermore, in terms of refueling (hydrogen) time, it offers performance with a value similar to the refueling times in conventional motor buses compared to battery-electric buses (fast charge concept). Finally, due to the electric motors used in the propulsion systems, the acceleration performance of the buses also increases significantly compared to the conventional propulsion systems (diesel engines).
In this paper, the fundamental parameters based on Life-cycle Cost Analysis, which is another crucial evaluation factor to be considered for public transportation systems, are included for the proposed bus concepts. These are purchasing, necessary infrastructure installation, and bus operating costs. In this context, given their widespread use, unit costs for battery-powered electric buses can be disclosed. However, the unit costs for fuel cell hybrid-electric buses have not yet reached the expected maturity levels. Performance results and costs obtained from fuel cell hybrid-electric buses, which are generally put into use within the scope of EU projects, are an important reference in this context. Also, Ballard, one of the most important fuel cell manufacturers in the world, states that the average energy consumption value for fuel cell hybrid-electric buses, including auxiliary systems (air conditioning, heating, lighting, etc.), is 9 kg H2/100 km (Hua et al., 2014). In the same token in the CHIC project, which followed the operation of 54 fuel cell buses in Europe and Canada between 2010 and 2016, it was stated that the average fuel consumption value for the reference 12 m fuel cell hybrid-electric bus was similarly measured as 9 kg H2/100 km (Eudy & Post, 2017).
Based on the scenarios foreseen for public transportation systems, it is expected that the purchasing costs of 12 m solo fuel cell hybrid-electric buses will reach approximately 400,000-450,000 €, while the articulated ones will reach 580,000-630,000 € in 2030 (Stolzenburg et al., 2020).
THE MAIN OPERATING COSTS FOR BUS CONCEPTS ON MODEL
There are some basic elements of the use of fuel cell hybrid-electric buses. These aspects must be considered by public transport authorities/operators. It is recommended to start with a small fleet and gradually increase the number of vehicles. Authority /operators must adapt to the new generation vehicle technology they will use and determine the right concept in line with business needs. This is important in terms of cost and operational continuity. Additionally, the training of bus drivers and maintenance staff enhances efficiency (Topal, 2023).
In this study, fuel cell hybrid-electric buses will be assessed as an alternative to battery-electric buses, which have gained prominence in today's sustainable public transportation approach. The technology maturity level of both concepts has demonstrated its effectiveness in the field of public transportation worldwide and can meet the necessary requirements for the zero-emission approach (Tank-to-Wheel). Also, there are disadvantages in both concepts (Ballard, 2023).
The initial purchase costs of battery-electric buses are lower than fuel cell hybrid-electric buses. However, it offers limited operating performance for different operating conditions. Battery-electric buses cannot meet the operational performances of internal combustion engine buses, which are currently widely used, especially for long or harsh conditions routes. In addition, the efficiency of the batteries decreases in different climatic conditions (summer/winter weather conditions).; Meanwhile, on-route charging infrastructure that is sometimes difficult to deploy and implies additional costs becomes essential to meet range requirements. In this context, fuel cell hybrid-electric buses offer flexible operation options for public transportation authorities/operators and have the potential to directly replace the currently used diesel/CNG buses for the 100% zero-emission option (Well-to-Wheel). Thanks to the fuel cell used, it maximizes the passenger capacity without increasing the vehicle's gross weight (compared to high-capacity batteries). For the analyses conducted in this study regarding the fuel cell system, the reference unit, which includes operational and infrastructure installation costs, was derived from the Fébus project. Fébus is the world's first Bus Rapid Transit (BRT) project to utilize fuel cell hybrid-electric buses. Additionally, performance results and unit costs obtained from significant projects conducted in America and Europe were also used for comparison.
The energy consumption of buses used in public transport depends on several factors, including load, weather conditions (heating system on/off), traffic, city terrain, vehicle dynamics, etc.
However, the energy consumption could vary for the same battery-electric buses with the same length, capacity, load condition, etc. This could be attributed to traffic conditions (or terrain) from one line to another or from one city to another. Weather conditions were also considered. It was found that some of the battery-electric buses consume an extra 64% of power in winter times due to the heating process. For an 18 m battery-electric bus, the average energy consumption for 10-month of operation period is around 1.63 kWh/km (Al-Saadi et al., 2022). According to the report by Hee the articulated battery-electric buses with a length of 18 m, Bozankaya Sileo and Solaris, were tested in three days of operation in Bonn. The unit energy consumption measurement with heating off, but air conditioning on, ranged between 1.65-1.84 kWh/km. According to these data, a range between 190 and 210 km can be covered by an 18 m battery-electric bus with 350 kWh of battery capacity (He at al., 2019).
On the other hand, the data presented is from AC Transit, the public transportation company operating in the United States, for fuel cell hybrid-electric buses. The fuel cell hybrid-electric buses are commercially used by AC Transit. It was reported between 2013 and 2016; 12 fuel cell hybrid-electric buses consumed an average of 3.02 kWh/km of energy. It was also reported that the average fuel cost is 0.89 $/km while the maintenance & repair costs of buses are in the range of 0.15 to 2.98 $/km on average 0.59 $/km. Another operator which is SARTA, has a fleet of 5 fuel cell hybrid-electric buses. It has an average fuel efficiency of 2.66 kWh/km, a fuel cost of 1.25 $/km (hydrogen), and a maintenance & repair cost of 0.21 $/km (according to data obtained between 2016-2019). In addition, there are 4 fuel cell hybrid-electric buses in the Sunline Transit. Based 2014-2016 data for the mentioned fleet, 3.14 kWh/km was reported for energy consumption, 0.88 $/km for fuel cost, and 0.26 $/km for maintenance & repair cost (Deliali et al., 2021). In general, changes are observed in the cost of hydrogen fuel in current applications, depending on the production methodology and amount. Differences in the methods used in basic categories such as hydrogen use, production and transportation vary between 4.52 $/kg and 23.5 $/ kg among public transportation companies. In this context, hydrogen costs for SunLine are 7.03-9.34 $/kg and for AC Transit, 7.50-7.68 $/kg, which are the oldest fleets by fuel cell hybrid-electric buses (Deliali et al., 2021). According to data from SunLine Transit and AC Transit, planned maintenance & repair costs are in the range of 0.06-0.30 $/km on average. It is stated that this value can increase to 0.24-0.61 $/km with additional costs derived from unplanned maintenance (Eudy & Post, 2017). It is also stated that while the buses are under warranty, their total maintenance costs are at the lowest level (approximately 0.25$/km). The general average is 0.46 $/km of maintenance & repair cost when the relevant businesses are taken as reference - based on the USA (Eudy & Post, 2017).
In Europe, it is stated that with the HyFLEET-CUTE project, 2.5 Million km was covered between 2003 and 2010 with 30 fuel cell buses in 10 cities (Pederzoli et al., 2022). Similarly, it is known that with the CHIC project, 26 fuel cell hybrid-electric bus public transportation companies are currently operating in 5 countries HyFLEET: CUTE Project, 2010). In addition, with the V. LO-City project, 14 fuel cell hybrid-electric buses are used in 3 countries (Italy, Scotland, and Belgium). Also, HyTransit is another program that provides 6 fuel cells hybrid electric buses (Ballard, 2022b). As can be seen, most of the projects carried out in Europe have been rexecuted with EU project support.
In line with the data obtained, the energy consumption (fuel) efficiency of the buses was obtained according to the propulsion system. Accordingly, it varies between 2.40 - 4.22 kWh/km for fuel cell hybrid-electric buses; 3.83-6.03 kWh/km for diesel buses (Deliali et al., 2021). In addition, the average hydrogen consumption value for 18 m fuel cell hybrid-electric buses is 9kg/100km in the Fébus Project which is the BRT system consisting of Europe's first fuel cell hybrid-electric buses. Finally, the planned maintenance & repair cost of buses is 0.72 € per km. It was stated that a 15-year full maintenance contract was signed, and these costs included the training to be provided for 2 years, the supply of spare parts, and the necessary special equipment.
Another important investment cost for fuel cell hybrid-electric bus concepts is that of refueling stations and facilities. It needs a different infrastructure in special conditions. AC Transit has reported that it has realized a budget of approximately 10 M $ for the cost of the hydrogen refueling station, which has a daily hydrogen production capacity of 600 kg, suitable for use in both cars and buses (Eudy & Post, 2014). Similarly, SARTA, reported that it spent 2.2 M $ for the installing of a new hydrogen refueling station with a capacity of 300 kg per day (Sokolsky, 2016). Finally, there is an electrolyzer with a hydrogen production capacity of 174 to 268 kg in 24 hours at the hydrogen refueling station, which is a "green" concept until the production and storage structures in Fébus. It has been stated that it has the capacity to store a 3-day reserve and 4.5 M € have been spent for the establishment of the station (Despaux, (2019). Furthermore, the comparison of diesel, fuel cell hybrid-electric, and battery-electric bus concepts for rubber-tired public transportation systems is included, considering various aspects. In this context, Table 2 is presented based on the evaluation criteria established for 100% zero-emission and operational compliance using the pros and cons method.
COMPARISON OF BATTERY-ELECTRIC BUSES AND FUEL CELL HYBRID-ELECTRIC BUSES UNDER REAL OPERATING CONDITIONS
Different climate, road, and travel conditions are considered as performance indicators in the fundamental comparisons between battery-electric and fuel cell hybrid-electric buses to be adopted by public transportation operators/authorities. Moreover, achieving longer-range values and minimizing the necessary infrastructure costs are of the utmost importance.
In the evaluations, it is recognized that battery-electric buses can be charged on their routes (at stops) using fast charging concepts, or overnight charging can be implemented with slow charging concepts at depots. In this context, battery-powered electric buses with the slow charging concept implemented at depots must have sufficient battery capacity to complete their designated routes. When buses are not in service, during their inspection (usually at night), charging processes take place depending on the charging station's power, energy infrastructure, and battery capacities. Flexibility can be achieved in the public transportation service routes by incorporating battery-electric buses into this concept. However, due to climate, road, and travel conditions, battery-electric buses may encounter certain limitations in terms of the range they can offer. Indeed, increasing battery capacities to enhance the range value can result in an increase in the total vehicle weight, leading to inefficiencies in unit energy consumption. This also reduces the passenger carrying capacity. Electric buses adhering to the fast-charging concept can operate efficiently by timely charging in short periods along their routes, either at stops or in designated areas at the beginning or end of the line. Charging times may vary depending on the charging station power and battery capacity. Battery-electric buses suitable for the fast-charging concept have low battery capacity. Therefore, their fixed battery load is reduced, as their routes are directly linked to routes where charging stations are deployed. Due to their low battery capacity, they need to be charged in shorter time intervals. Considering the operational factors, performance, and other business requirements, it can potentially cause limiting effects. Another battery electric bus concept that can be evaluated in this context is that of trolleybuses. For the rubber-wheeled bus concept, powered by overhead catenary lines, operation can also be provided with small-capacity batteries in regions where there is no catenary line. However, a significant amount of infrastructure (catenary line) investments is required for trolleybuses and providing services on fixed routes poses significant disadvantages in this sense.
Table 3 shows the primary alternatives for the zero-emission approach in public transportation enterprises, encompassing battery capacities, charging times, range values, and the variation in total vehicle curb weights for battery-powered electric buses with various battery capacities of 12 m battery-electric buses.
Table 3 Different Parameter's Impact on the Main Range Values of Battery-Electric Buses
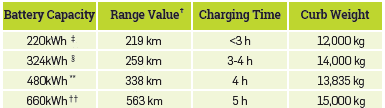
† Range is based on Altoona testing conditions
‡ The Proterra Catalyst® 40-Foot Transit Vehicle. (2017, April 18). Retrieved from http://www.proterra.com/products/catalyst-40ft/
§ K9 Electric Bus. (2017, April 18). Retrieved from http://www.byd.com/usa/bus/k9-electric-transitbus/
** Xcelsior Electric. (2017, April 18). Retrieved from https://www.newflyer.com/buses/zero-emissions/xcelsior-electric-bus
†† The Proterra Catalyst® 40-Foot Transit Vehicle. (2017, April 18). Retrieved from http://www.proterra.com/products/catalyst-40ft/
The above range values given above are based on ideal conditions for 12 m solo buses. It is reported that the batteries used in the measurements are at the beginning of their life cycle, the buses operate on a flat route without slopes, they have only seated passengers (no standing passengers), and performance measurements are performed without cabin heating or cooling. Under the specified conditions, the energy consumption of the propulsion system for battery-electric buses is 1 - 1.4 kWh/km. The charging station is 1.1 to 1.5 kWh/km over the mains supply (AC system) and 1.3 to 2 kWh/km is consumed in addition to the current consumptions for air conditioning (for heating/cooling systems) (Ballard, 2022a).
On the other hand, fuel cell hybrid-electric buses, which are considered to be an important alternative. A fuel cell module is incorporated into the vehicle system architecture. This approach enables the drive battery to maintain an optimal charge level. By combining both the fuel cell and battery in a hybrid system, an efficient and 100% zero-emission public transportation concept has been proposed, free from range or route constraints. During the electrochemical reaction, the power module of the fuel cells in buses produces electrical energy while releasing water and heat as byproducts. Additionally, the heat released during the electrochemical reaction can be utilized. The fuel cell module can be integrated into the engine room of the bus (at the rear of the vehicle - similar to conventional buses), or on the roof. Hydrogen storage tanks are typically located on the roof of the bus, similar to the fuel tanks in CNG (Compressed Natural Gas) buses. Integrating the fuel cell module into the system architecture leads to a significant reduction in the size of the battery pack. Moreover, it contributes to a reduction in the additional costs (compared to battery-electric buses) incurred from the need to replace batteries at certain intervals over the bus's total lifespan. By hybridizing the fuel cell system with batteries, the fuel cell can operate at a relatively slower speed, leading to a substantial increase in efficiency. Moreover, this hybridization enables a constant power output while preventing deep discharges of the batteries, which enhances overall performance and prolongs battery life.
Decreasing the total weight of fuel cell hybrid-electric buses compared to battery-electric buses enhances energy efficiency.
Hence, it is possible to accommodate more passengers. The range of fuel cell hybrid-electric buses varies depending on the amount of hydrogen stored in the bus, as shown in the table. The performance data of 12 m solo buses with different hydrogen capacities are provided in Table 4.
Table 4 Different Parameter's Impact on the Range of Fuel cell hybrid-electric Buses
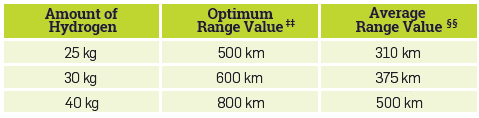
†† Characterizes as Ideal Range conditions, assuming a hydrogen consumption rate of 5 kilograms per 100 kilometers
§§ It is characterized as Average Range conditions, assuming a fuel consumption of 8 kilograms per 100 kilometers
The range values in the table are based on smooth road conditions, when the auxiliary power systems are off and the total system efficiency (for the drive group) is 90%. It is also stated that fuel cell hybrid-electric buses consume 7.1 to 8.9 kg/100 km of hydrogen under different climate and route conditions (Eudy & Post, 2020).
Another study, conducted by Ballard, evaluated the performance results of fuel cell hybrid-electric buses and battery-electric buses. According to the e-SORT (Standardized On-Road Test) test protocol proposed by UITP, the performance results of two 12-meter buses (battery-electric and fuel cell hybrid-electric buses) were measured in urban, inter-urban, and mixed conditions. Based on the test results obtained from the e-SORT test cycles, a 20-minute driving profile at an average speed of 15.4 km/h was replicated to simulate a full day's operating conditions. The technical specifications of the buses used in this case study are shown in Table 5 (Ballard, 2021a).
Based on the performance measurements of the battery-electric bus, it has been reported that it achieved a range of approximately 210 km within a one-day operating cycle lasting 13.6 hours. This was achieved with a battery capacity of 200 kWh on a smooth route, and without using auxiliary loads. Furthermore, it has been acknowledged that the acceptable discharge depth for the propulsion battery ranges from 90% to 10%, and the usable battery capacity is limited to 80%. On the other hand, the range value of the battery-electric bus decreased in harsh route conditions and when auxiliary system loads were included. It is reported that while the total auxiliary system loads result in an increase of 20 kW, the maximum increase in range occurs in road conditions with a 1,050 m long hill having an 8.6% incline, primarily due to the traction system. Considering these conditions, it has been demonstrated that the battery-electric bus can cover a distance of 73 to 78 km without the need for recharging in a 5-hour period (Ballard, 2021b). In addition, performance measurements based on the same conditions were conducted with a fuel cell hybrid-electric bus. The fuel cell in the system operates as a serial hybrid structure, providing the necessary charging for the propulsion battery. The proposed system design ensures that the bus loads are met under peak conditions without the need to recharge the batteries, thanks to the inclusion of the fuel cell and its charging capabilities. Therefore, the need for a charging infrastructure is eliminated with this system design. The fuel cell system utilizes the energy generated from the fuel cell to maintain the battery charge level within a specific range, ensuring that the battery remains at its optimal charge level. These results indicate that fuel cell hybrid-electric buses achieve better performance under the specified conditions. Fuel cell hybrid-electric buses offer a technological solution that can directly replace the currently widely used diesel buses. It introduces a concept that provides businesses and operators with a flexible and zero-emission option for sustainable public transportation systems. Performance results under real operating conditions, based on 12 m fuel cell hybrid-electric and battery-electric buses used in public transportation systems, offer a significant alternative for selecting the appropriate concept for BRT systems, which are the focus of this study (Ballard, 2021b).
THE WORLD'S FIRST BRT LINE WITH FUEL CELL HYBRID-ELECTRIC BUSES: FÉBUS PROJECT
It is stated that the Fébus project demonstrates the vision of efficient and new zero-emission mobility for public transport in France. In the project, which started in the south of France in 2015, fuel cell hybrid-electric buses are highlighted as the primary approach for operation, particularly on the BRT (Bus Rapid Transit) line. Since 2019, a total of 8 Van Hool Exqui. City FC 18 m, fuel cell hybrid-electric buses have been operating on a 6 km Metrobus line in Pau, France, providing public transportation services. The system is operated by STAP (Société de Transport de l'Agglomération Paloise).
The 8 units made up of 18 m long buses manufactured by Van Hool used in the project are designed with a Ballard's FCveloCity®-HD 100 kW fuel cell. It is said that these buses are suitable for the BRT system and can travel approximately 300 km in one trip with one refueling. Figure 3 shows the fuel cell hybrid-electric buses used in the project. As of December 2019, the fleet has covered a total distance of more than 810,000 km. The buses have a passenger capacity of 145 (32 seating areas). In addition, the hydrogen supply process (hydrogen supply and storage service) is provided through ENGIE and GNVERT companies. ITM POWER designed the hydrogen production systems using electrolysis and performed the production and distribution of the green hydrogen needed for the project through electrolysis.
Indeed, the power supply is also sourced from renewable energy systems. Consequently, the entire process from hydrogen production to consumption by buses has been established as a 100% zero-emission public transportation solution, ensuring that well-to-wheel emissions are minimized. It is stated that the installation of an 8-bus fleet in the Fébus project was financed entirely by the EU project supports, with 3 buses funded by the 3Emotion project and 5 funded by the Jive2 project. The Fébus project had a total investment of 74.5 M € and the biggest cost within the scope of the project is 50 M € for construction activities. As part of the project, various construction works were undertaken, including the construction of stations and landscaping facilities. This involved the renewal of the 10 km BRT line, the construction of a 6 km bicycle path, a 5 km additional pedestrian path, afforestation covering 6 hectares along the entire route, and the arrangement of a square. Furthermore, it was mentioned that 10 M € was allocated for the procurement of buses, and an additional 4.5 M € was invested in the installation of the Hydrogen Supply Station (Despaux., 2019). These costs serve as reference for vehicle purchase and refueling station installation costs in the analyses conducted hereunder.
In the analyses conducted by STAP within the scope of the Fébus project, it is mentioned that the battery-electric bus concept, as an alternative to fuel cell hybrid-electric buses, was also assessed to meet the operational requirements of existing operations. In the aforementioned analyses, it was found that the operation provided by 8 fuel cell hybrid-electric buses with hydrogen refueling in the depot could be achieved by using 10 fast-charging and 14 slow-charging battery-electric buses with similar capacity (Fébus, 2022).
There is also a specially designed hydrogen refueling station within the scope of the project. The "green" hydrogen obtained for the station, which includes production and storage structures, a 100% zero-emission perspective is offered from the well to the wheel. The station, designed for the storage and refueling of hydrogen, has an electrolyzer capable of producing 174 to 268 kg of hydrogen per 24 hours. The facility has a capacity to store reserves for 3 days. With the refueling processes to be carried out during the night inspection of the buses (based on 9 to 12 kg of hydrogen consumption per 100 km), it has been learned that the buses have a fuel tank that will provide a range of at least 240 km, and that refueling takes 30 minutes. Figure 4 below shows the concept of the hydrogen supply and storage facility (Ballard Power Systems, 2021).
According to the information obtained in the interview conducted with the project manager of Fébus, Ms. Lucie KEMPF, in the Fébus project, the water supply required for the electrolyzer of the station providing H2 refueling for the 18-meter long, 8 fuel cell hybrid-electric buses is sourced from dams located near the city of Pau, which is approximately 40 km away. In the mentioned project, the average hydrogen consumption value is 9 kg per 100 km. Additionally, a maintenance fee of 30,000 € per month was paid for the fuel station operating cost under the 15-year "all-inclusive" contract signed with ENGIE (station operator).
Lastly, it has been reported that a 15-year full maintenance contract (Vanhool) was entered into with a cost of 0.72 € per km for the planned maintenance and repair of the buses. These costs include training for 2 years, the supply of spare parts, and the necessary special equipment.
4. THE FINANCIAL MODEL FOR 100 % ZERO EMISSION CONCEPT ON ISTANBUL METROBUS LINE
This study is based on an extensive literature review and data collected from real-world field conditions. Indeed, the study includes evaluations of diesel, battery-electric, and fuel cell hybrid-electric bus concepts. The financial analyses conducted in this study involved obtaining the necessary investment, infrastructure installation, and operating costs related to the relevant bus concepts. The costs used as the basis for the analyses, conducted in accordance with the Payback Period Method, and based on a 15-year use period for public transportation systems, are shown in Table 6.
The main data related to fuel cell hybrid-electric buses and their infrastructure facilities used in the analyses were obtained from the Fébus Project, which is the world's first BRT System to implement fuel cell hybrid-electric buses. Additionally, operational conditions, performance results, and costs associated with diesel buses were taken from the Metrobus System in Türkiye for comparison in the analyses. Similarly, the operating data and costs for battery-electric buses were obtained from the Municipality of Elaziğ in Türkiye for the analyses.
According to the foregoing, in 2021, the IETT General Directorate conducted a tender for the purchase of 100 new 18-meter buses with internal combustion engines (diesel) that are single articulated. These buses were purchased for the Metrobus line. As part of tender number 2021/389857, the first batch of deliveries was made in 2022 for the purchasing (100 new 18-meter single articulated diesel buses) for the Metrobus line. The contract was signed on 08.09.2021, with a total cost of 61.3 M €. According to the technical specifications within the scope of the tender, it is evident that the 3-year maintenance & repair cost is included in the purchase. In the analyses carried out, the unit costs that are the basis of this tender and the fuel supply station installation costs at the capacity foreseen for the diesel bus concept are included (EKAP, 2021; EKPA, 2016) . The fundamental costs of the battery-electric bus concept were derived from the contract signed by the Municipality of Elaziğ with tender registration number 2017/111661 on 16.05.2017. It is evident that the total contract price of 13.1 M € includes 15 battery-electric buses of 18 m (single articulated), 15 charging stations, and the 2-year maintenance & repair costs for the buses (EKAP, 2017). Lastly, for the fuel cell hybrid-electric bus concept, the Fébus Project is used as a reference for the bus and infrastructure costs. It is reported that the 18 m (single articulated) buses procured within the scope of the project have a total investment cost of 10 M €, and the installation cost of the Hydrogen supply station amounts to 4.2 M € (Fébus, 2022b).
For financial analyses purposes, the labor costs associated with the maintenance and repair of the bus concepts and related infrastructure components, have been carefully evaluated and considered. A specific "escalation parity" was introduced for this study. This parity enables an effective comparison where the minimum wages in Turkey, where the study is focused, are based on the minimum wages in France, where the Fébus Project is implemented. Hence, it is considered that efficiency has been achieved in such analyses. For the specified periods at the time of this study, the minimum wage in Turkey is 4,253.40 TL, and the minimum wage in France is 1,603.00 Euros. Based on this parameter, known as EP (escalation parity), an effective comparison of labor costs, which constitutes a significant expense in the maintenance & repair processes for buses and charging stations, is made. The EP parameter is set at 6.07 (Official Journal, No. 31692, 2017).
Diesel bus concepts have standardized approaches to maintenance and repair unit costs, whereas for battery and fuel cell hybrid-electric buses, there is no established standard approach. It is considered that the lifespan of the battery should also be considered in the long-term contract-based approaches for battery-electric buses, which provide the flexibility to operate under different concepts. In this study, analyses were conducted based on a 15-year average lifespan for buses in rubber-tired public transportation systems. Over this time, the propulsion battery, which is the main powertrain component of battery-electric buses, may need to be replaced. Maintenance and repair costs for electric buses may become challenging to maintain the determined performance conditions, especially after the first 5-year period. Renewing the powertrain battery at least 2 times within the stipulated period leads to a significant increase in maintenance and repair unit costs (Topal, 2023). It has been reported that the maintenance & repair process under warranty continues for the electric buses in Elaziğ Municipality which are referenced in this study (EKAP, 2017). There is no long-term recorded usage data available for other electric buses used in Turkey. In the analysis, the maintenance & repair unit costs for battery-electric buses were obtained from the literature. For diesel buses, the current operational data of IETT was used, while for fuel cell hybrid-electric buses, the current operational data of STAP was utilized. Details are shown in Table 6.
Fuel/energy unit costs, which are another significant operating expense, were also obtained from real-world conditions as part of the analyses. The unit price of fuel (diesel) for diesel bus concepts is 1,35 Euro/lt , the price of AdBlue 1,37 Euro/lt and the price of oils (transmission and engine oils) 0,014 Euro/km based on the supply conditions provided by the IETT. For the energy consumption unit price for the battery-electric bus concept, the electricity unit price provided by the IETT. It has been included in the calculations as 0,094 kWh/Euro, including taxes, based on the industry tariff. Finally, no cost was considered for Hydrogen used in fuel cell hybrid-electric buses in the calculations, following the approach of the Fébus Project. In the Fébus Project, Hydrogen production is provided on-site through the infrastructure installations, thus eliminating the need for external hydrogen supply (H2). In this context, it has been mentioned that the electrical energy required for the electrolyzer used in the hydrogen refueling station is supplied from a nearby hydroelectric power plant (approximately 40 km away). This approach showcases the use of "green hydrogen" as a fully demonstrated solution in the Fébus Project (Despaux., 2019).
Finally, the necessary infrastructure installations specific to the bus concepts included in the analysis are designed with hydrogen storage and replenishment facilities for fuel cell hybrid-electric buses. The Hydrogen refueling station is equipped with an electrolyzer capable of producing 174 kg to 268 kg of hydrogen per 24 hours and it has a capacity to store a 3-day reserve of hydrogen. For battery-electric buses, the charging process is completed in 3-4 hours with 8 charging stations (with 125 kW charge power). Also, the diesel bus concept is based on the supply of 4 dispensers (with 8 fuel guns) and a fuel storage tank that can store a 3-day reserve. The charging stations, hydrogen and fuel refuelling stations for different bus concepts are shown in Figure 5 below.
In the analysis, real data was used, and the number of vehicles in the Fébus Project, which is the first and only BRT system based on the 18 m (single articulated) fuel cell hybrid-electric bus concept, served as the main reference. Relevant calculations were made on a total of 8 fleets, including diesel, battery-electric, and fuel cell hybrid-electric buses. Bus tendering and infrastructure investments for rubber-wheeled public transportation companies are predominantly funded by local governments. Public transportation businesses operating under the non-profit model, in accordance with the social welfare principles, have been significantly affected by the recent economic conditions. With rising operating costs, especially in metropolitan municipalities, public transportation services under the non-profit model are facing challenging financial conditions. The rising operating costs, particularly in fuel prices, require sustainable and innovative approaches. To enable public transportation service providers to make the right investment decisions and determine the suitable solutions based on their needs, it is essential to adopt a holistic perspective in the projects.
The main focus of this study is to determine the payback period for the total investment value associated with vehicle purchases and infrastructure investments for three different bus concepts (diesel, fuel cell hybrid-electric, and battery-electric). This analysis takes into consideration both operating costs and income to assess the feasibility of each concept. The financial perspective presented is considered one of the key determining criteria for public transportation companies in terms of sustainability. The Payback Period Method was chosen for financial evaluation in the study. Criticims on this method includes its not considering the time value of money and the economic life of the investment, as well as not taking into account the net cash inflows that will be generated after the payback period. This may eliminate the opportunity to prioritize long-lasting and strategically important investments for the business. However, the method also has its advantages, such as being easy to understand and implement, allowing for simple comparison of different investment options, reducing the risk associated with the investment, and assisting businesses with liquidity issues to solve their problems. These are some of the strengths of the payback method. Using the results obtained, a comparison of the total fixed investment and expenses required for diesel, fuel cell hybrid, and battery-electric bus concepts is indexed to the income generated. The detailed analysis results are provided in Table 7.
The Equation 1 below is the primary formula of the payback period method for financial valuation. In Equations (1);
"PP" payback period time (year),
"Mo" bus purchase cost,
"G" average annual revenue per bus,
"IM" represents the operating cost per bus on an annual basis.
Based on this main methodology, four fundamental approaches have been presented. The Payback Period Method, as used in this analysis, concentrates solely on passenger ticket income as the main source of revenue. The calculations consider real conditions, including operating and installation costs of the metrobus lines in Istanbul and Pau, as the foundation for evaluating the various bus concepts. The analysis considers various factors, such as infrastructure installation costs, fuel expenses, and maintenance & repair operating costs for each bus concept.
The first of these are the formulas found in Equation (2) and Equation (3), referred to as the Payback Period (Emission Incentive). In the "Payback Period (Emission Incentive)" calculations, Equations (2) and (3) introduce an additional parameter. In this section, the Well-to-Wheel approach is used with the aim of offering incentives for fuel cell hybrid-electric buses and battery-electric buses. These incentives involve tax reductions, specifically 1% SCT (Special Consumption Tax) + 9% VAT (Value Added Tax) for fuel cell hybrid-electric buses and 1% SCT + 4% VAT for battery-electric buses, in comparison to diesel buses. Indeed, this approach reduces the initial investment cost for these zero-emission bus concepts.
"PPEI_FC" payback period time for fuel cell hybrid-electric bus concept with emission incentive included (year),
"PPEI_BE" payback period time for battery-electric bus concept with emission incentive included (year),
"Mo" bus purchase cost; while 0.90 coefficients for fuel cell; 0.95 coefficients for battery-electric bus,
"G" average annual revenue per bus,
"IM" represents the operating cost per bus on an annual basis.
Additionally, another evaluation parameter in the model is defined as "Payback Period (Comparative)". In this method, an approach is presented based on the additional initial investment costs incurred for fuel cell hybrid-electric and battery-electric bus concepts, compared to the reference diesel bus concept. The focus is on comparing the operating expenses and purchasing cost specific to each selected bus configuration. Absolutely, the calculations involve an approach that considers the investment costs unique to fuel cell hybrid-electric and battery-electric buses. These costs are added to the base value, represented by the initial investment cost of the diesel bus concept. This enables a comprehensive comparison of the overall investment costs among various bus concepts.
In Equations (1.3) and (1.4);
"PPC_FC" payback period time for fuel cell hybrid-electric bus concept comparative with diesel concept (year),
"PPC_BE" payback period time for battery-electric bus concept comparative with diesel concept (year),
"MFC" bus purchase cost for Fuel cell hybrid-electric bus concept,
"MBE" bus purchase cost for battery-electric bus concept,
"MD" bus purchase cost for diesel bus concept,
"G" average annual revenue per bus,
"IM_FC" operating cost per bus on an annual basis for Fuel cell hybrid-electric bus concept,
"IM_BE" operating cost per bus on an annual basis for battery-electric bus concept,
"IM_D" operating cost per bus on an annual basis for diesel bus concept
Finally, a hybrid approach is presented, which considers the expected support and incentives, as well as the purchase cost differences for the reference diesel bus concept. According to the approach named "Payback Period (Comparative and Emission Incentive Included)", the goal is to mitigate the existing disadvantage of the fuel cell hybrid-electric bus concept in terms of the initial purchase cost when compared to diesel buses. This is achieved by considering both comparative factors and emission incentives in the analysis, enabling a more comprehensive evaluation of the financial feasibility and advantages of adopting the fuel cell hybrid-electric bus concept.
Table 7 provides a detailed overview of the analysis results for all the approaches considered as the basis of the model. According to the Payback Period method results, the payback periods are as follows: 31.50 years for the diesel bus concept, 13.26 years for the fuel cell hybrid-electric bus concept, and 9.77 years for the battery-electric bus concept.
Table 7 Analysis Results
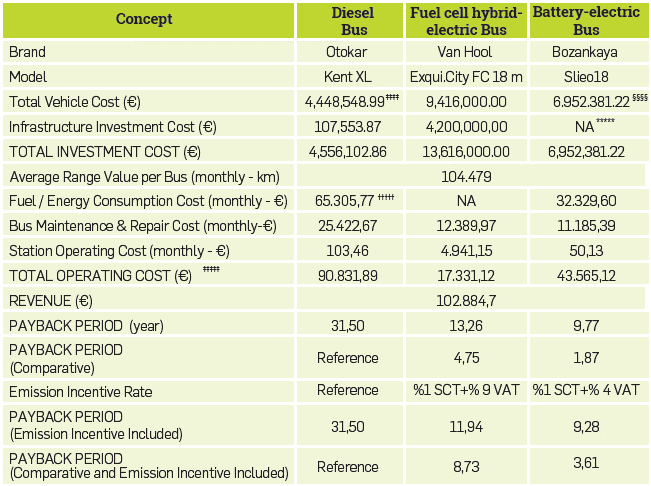
‡‡‡‡ Bus unit costs include a 3-year maintenance & repair guarantee as per the contract.
§§§§ Bus unit costs include a 2-year maintenance & repair guarantee as per the contract.
***** 15 Charging Stations are included in the tender price.
††††† Adblue, engine and transmission lubricant oils are included for diesel concept
‡‡‡‡‡ It is the value reduced to 8 buses and normalized over the annual average value.
According to the second proposed approach, which is described as Payback Period (Comparative), the cost of the reference diesel bus concept is kept constant. The additional costs incurred for the fuel cell hybrid-electric bus concept compared to the diesel bus concept are considered as the initial investment cost. This approach allows for a direct comparison of the investment required for adopting the fuel cell hybrid-electric bus concept instead of the diesel bus concept. In the calculations made in this approach, for the case where the fuel cell hybrid-electric bus concept is preferred over the diesel bus concept, the purchase cost of diesel buses is kept constant, and the additional costs required for fuel cell hybrid-electric buses are taken into consideration. Similarly, operating costs are calculated based on the difference between the fuel cell bus concept and the diesel bus concept. The aim is to evaluate the cost savings and benefits that can be achieved by adopting the fuel cell bus concept in terms of operational expenses compared to the conventional diesel bus concept. Through the consideration of these differences, a thorough analysis of the financial implications and advantages of selecting the fuel cell bus concept can be conducted, facilitating a more informed decision-making process.
The main objective of this comparison is to assess the financial implications of choosing the fuel cell hybrid-electric bus concept instead of the diesel buses, by considering the incremental costs involved in adopting the more environmentally friendly technology. In this context, the focus is on comparing the financial implications of opting for the fuel cell hybrid-electric bus concept instead of the diesel buses by considering the incremental costs involved in adopting the greener technology. This time, the result is 4.75 years. From the same perspective, if the battery-electric bus concept is chosen instead of the diesel bus concept, the result is 1.87 years. This approach is referred to as "PAYBACK PERIOD (Comparative)" in Table 7. On the other hand, one of the important results revealed is the emission rates of the bus concepts in question. In this context, analyses were repeated in accordance with a separate parameter (based on this study) according to the Well-to-Wheel and Tank-to-Wheel approaches. It is envisaged to provide incentives with a tax reduction of 1% Special Consumption Tax (SCT) + 9% Value Added Tax (VAT) for fuel cell hybrid-electric buses and 1% SCT + 4% VAT for battery-electric buses, against diesel buses, which are preferred due to their initial investment cost, although they pose a disadvantage especially in terms of emissions. According to the analysis results described as 'Payback Period (Emission Incentive)' in Table 7. In this case, the first purchase costs of buses are based on tax deduction and providing incentives. The results for the diesel bus concept remain the same as it serves as a reference. The obtained results are 11.94 years for the fuel cell electric bus concept and 9.28 years for the battery-electric bus concept, eventually, in the "Payback Period (Comparative and Emission Incentive)" model in Table 7. Also, the calculations were renewed together with the tax incentives based on the previous approach. The results are the following: if the fuel cell hybrid-electric bus concept is preferred instead of the diesel bus concept, it corresponds to 8.73 years, and if the battery-powered electric bus concept is preferred, the results of 3.61 years have been achieved.
You can see comparison results of the analysis of the Payback Period Model in different concepts in Figure 6. Analyses were performed with the data obtained, considering the current operating conditions for the Istanbul Metrobus line. Despite the reduced number of trips due to the pandemic conditions, calculations were made for 104,479 km on a monthly average per bus. Accordingly, the total operating costs are calculated as 90,832 Euro/month, 17,331 Euro/month and 44,086 Euro/month for diesel, fuel cell hybrid and battery-electric bus concepts, respectively. Similarly, travel revenues reduced to vehicle basis were also included in the calculations to obtain the final results. Due to the distance-based tariffs and free/discounted trips applied on the Istanbul Metrobus line, a revenue cannot be calculated directly based on the number of trips.
CONCLUSIONS
The financial and operating costs of different powertrain systems of buses can vary significantly depending on a variety of factors, such as the specific make and model of the bus, the local cost of fuel or electricity, and the local climate and weather conditions. An alternative operation model for public transportation systems based on the bus rapid transit (BRT) system is discussed in this paper. In general, fuel cell hybrid-electric buses and battery-electric buses may have higher upfront costs compared to traditional diesel buses, but they may have lower operating costs over the long term due to the lower cost of fuel or electricity and the potential for lower maintenance costs. Zero-emission buses may also have lower operating costs, as they do not produce any emissions and may have lower maintenance requirements.
In the same vein, there are a variety of ways for governments to encourage the adoption of zero emission buses, such as offering financial incentives for bus operators to purchase zero emission buses, setting regulations or standards for the minimum number of zero emission buses that must be in operation, or investing in infrastructure such as charging stations or hydrogen fueling stations to support the use of zero emission buses. Rubber-tired public transportation systems are at the forefront of transportation-related emissions. In this respect, it increases the search for sustainable transportation solutions based on the 100% zero-emission approach for public transportation systems, which are mostly operated by local governments around the world.
In this paper, the fuel cell hybrid-electric bus concept is discussed as an alternative to the battery-electric buses, which are becoming more common in the world for public transportation systems, according to the real operating conditions. Pursuant to the results of the financial analyses conducted, the total cost of ownership components are compared in terms of technical, environmental, and financial aspects. According to the presented model, the results of the approach where the best outcomes are achieved from the "Comparative and Emissions-Induced" approach, the details of which were explained in the previous section, for the Payback Period Method finally used. In the event of using the fuel cell hybrid-electric bus concept instead of the diesel bus concept and providing incentives at the determined rate, the results were 8.58 years. Similarly, when the battery-electric bus concept was used instead of the diesel bus concept, the results were 3.29 years. Currently, as there are no incentive and support mechanisms based on the zero-emission approach in the public transportation sector on vehicle side; analyses were conducted relying only on a predictive approach rate hereunder. The intent is to raise awareness among decision-making mechanisms and public transport authorities.