1. Introduction
Postharvest losses do not only include physical losses in amount, but also a highly significant degradation of essential bioactive compounds and quality attributes in general. Hence, minimizing postharvest losses of perishable agricultural products throughout the whole value chain, from the farm to the table, is one of the key ways to alleviate poverty, increase food security food security, and improve nutrition [1].
This is how, currently, a higher percentage of consumers has become more aware of the importance of nutrition and is showing potential interest in convenience products with health benefits. In response to such, in the modern lifestyle, the market of fruit is on the rise globally. Not only as convenient foods and ready to eat, but because of their good nutritional and biological properties, which are considered a new type of promising functional foods as is the case of CP+FAC [2]. In the food industry, packaging materials and storage conditions are considered the last step in the product’s development to extend the conservation of dry fruit. During storage and distribution, dry products can experience a broad range of environmental conditions (temperature, humidity, light, and exposure to oxygen), which can trigger diverse changes from browning reactions that cause undesirable colors or other chemical reactions that can degrade important contents of FAC (polyphenols, carotenoids, and vitamins, among others), which is a particular concern for consumers, given that the nutritional value diminishes [1-3].
Few studies have reported on the evaluation of the degradation kinetics of FAC and, especially, in powdered foods during storage, ignoring the exact mechanism that causes the degradation, which is why the problem is generally solved by adding a higher concentration before processing, so that the recommended level is present at the end of its commercial life. Among the works found, these generally evaluate the effect of storage conditions, like type of packaging (with O2, without O2, vacuum, with N2), TA, tS, RH, presence of light and generally predict the shelf life of the product stored: Chávez-Servín et al., [4], in powdered infant formula; Pua et al., [5], with powdered jackfruit (Artocarpus heterophyllus); Frias et al., [6], with enteral formulas; Jiménez-Aguilar et al., [7], with blueberry powder; Moraga et al., [8], with grapefruit powder; Kha et al., [9], with gac oil powder; Tan et al., [10], with bitter melon powder; Islam-Shishir et al., [11], with guava powder; Muzaffar and Kumar [12], with powdered tamarind pulp; Udomkun et al., [1], in dry papaya chunks; Araujo-Díaz et al., [13], with powdered blueberry juice; Zorić et al., [14], with cherry powder; Mahmoodani et al., [15], with powdered whole milk; de Moura et al., [3], with Hibiscus sabdariffa L; Syamila et al., [16], with powdered spinach juice.
Thereby, the challenge for modern researches is not only to minimize the chemical degradation reactions, but to also maximize the conservation of beneficial nutrients during storage; hence, the object of this research was to assess the degradation kinetics during storage of vitamins (C, D3, and E), total phenols, and properties associated with the antioxidant activity of CP+FAC obtained through the spray drying (SD) technique.
2. Materials and Methods
2.1. Materials
The study used the Malayan dwarf coconut variety from the Colombian Pacific coast, and the CP+FAC was obtained through SD according to the methodology described in Lucas et al., [17]. The product was packaged with N2 and atmospheric air, using multilayered bags (Alico S.A.), laminated film (PET) thickness: 12 (m, aluminum foil: 8 (m, polyethylene sealant layer: 100 µm, grammage of 136.4 g/m2, water vapor barrier (<5 cc/m2 x 24 h x atm) and O2 (<5 cc/m2 x 24 h x atm). Storage was carried out in climatic chambers conditioned to 65% relative humidity, temperatures: 15, 25, and 35 °C and times: 0, 30, 60, 90, 120, 150, and 180 days.
2.2. Characterization of the CP+FAC
Vitamin C extraction was conducted according to the methodology described by Silva et al., [18], and vitamins D3 and E according to the methodology proposed by Ruiz-Ruiz et al., [19], modified by inclusion treatment through ultrasound (40 kHz) during 20 min, for the samples treated with hexane. Vitamin C quantification was performed through high-resolution liquid chromatography (H-RLC) (Shimatzu Prominence 20A), using a column in reverse phase (C18 - 5 µm, 4.6 mm x 250 mm), diode array detector, mobile phase: KH2PO4 0-02 M pH = 3.00 (Ortho-Phosphoric Acid 85%), flow: 1 mL/min, furnace temperature of 35 ºC, 244-nm wavelength and injection volume of 5 µL. Vitamins E and D3 were quantified using a column in reverse phase (C18 - 5 μm, 4.6 mm x 250 mm), diode array detector, mobile phase: acetonitrile/methanol/water (45.3/51.2/3.5), flow: 1 mL/min, furnace temperature of 40 ºC, and wavelengths of 285 and 265 nm, respectively [20].
Total phenol (TP) content was determined according to the methodology described by Zorić et al., [14], (mg Gallic acid/g CP+FAC); The DPPH free radical scavenging activity (mg of trolox/g CP+FAC) and ABTS (mg of trolox/g CP+FAC), was determined according to the extraction methodology proposed by Sridhar and Linton-Charles, [21] and quantification according to Casagrande et al., [22].
2.3. Degradation kinetics
The evolution of the FAC content, total phenols, and antioxidant activity in the CP+FAC was quantified in terms of retention percentage (Eq. 1) and modeling with zero - first - and second-order kinetics, described in Eq. 2, 3, and 4, respectively, where C 0 and C t are the values of the quality attribute evaluated at time 0 and t, with k being the degradation constant [14,23,24].
Additionally, the study evaluated the effect of temperature from the Arrhenius equation (Eq. 5), determining the activation energy (Ea) and the pre-exponential or frequency (A) factor, with T being the absolute temperature (K) and R the universal constant of gases (8.314*10-3 kJ/K* mol) [14, 23, 25]; where Ea was calculated from the slope of the graphic Ln (k) vs. 1/T.
2.4. Experimental design
The evaluation of the stability of the CP+FAC properties during storage was conducted by applying a design with sixth-* seventh-factor factorial arrangement with two independent variables: treatment and time, where treatment is defined as the combinations (temperature - packaging). The samples were stored in multilayered bags (Alico S.A.) and packaged in N2 atmosphere and with atmospheric air (Amb). The treatments applied were the following: 15 ºC-N2, 15 ºC-Amb, 25 ºC-N2, 25 ºC-Amb, 35 ºC-N2, and 35 ºC-Amb, and the storage times (tS): 0, 30, 60, 90, 120, 150, and 180 days. The dependent variables evaluated were: retention percentage of vitamins and antioxidants (%R): %R-VC, %R-VD3, %R-VE, %R-TP, %R-DPPH, and %R-ABTS.
Analysis of the results was performed by using Statgraphics XVI.I software, with analysis of variance (ANOVA) with multiple range test, 95% confidence interval, and the Tukey’s test of honest significant difference (HSD). The linear model generalized to relate the response to the independent variables was the following (Eq. 6):
3. Results and discussion
3.1. Initial content of FAC in CP and behavior throughout storage
The properties of the CP+FAC obtained through SD were as follows: humidity: 1.68±0.15%; aw: 0.17±0.02; solubility: 58.40±2.06%; Hygroscopicity: 8.36±0.55%; color: (L*: 79.45±0.90, a*: 1.50±0.13, b*: 9.50±0.45); wettability: 263.00±19.80 s, Peroxide index: 2.43±1.28 meqH2O2/kg fat; distribution of average particle size: (D10: 1.70±0.05 µm, D50: 8.46±2.09 µm, D90: 78.18±24.30 µm).
The proximal composition of the CP+FAC was: fat: 30.54±0.90%; protein: 4.07±0.49; total dietary fiber: 23.87±1.61%; ashes: 2.33±0.03%, and humidity: 1.68±0.48%, and the amounts of vitamins and antioxidants at time zero (0 days) were: vitamin C (VC): 2.11(0.10 mg/g of CP+FAC; vitamin D3 (VD3): 223.76(12.51 mg/g CP+FAC; vitamin E (VE): 0.20(0.01 IU/g CP+FAC; total phenols (TP): 29.7(7.6 mg Gallic acid/g CP+FAC, DPPH: 0.46(0.004 mg trolox/g CP+FAC and ABTS: 0.54(0.002 mg trolox/g CP+FAC.
The ANOVA had statistically significant differences (p <0.05) in all the dependent variables, with respect to tS, treatment (Temperature-Packaging), and the Treatment-tS interaction; in general, during 180 days of storage under the conditions described, significant losses occurred in all the treatments, both in vitamins as in antioxidants; with greater %R-Vitamins and Antioxidants at lower TA, and in treatments where the CP+FAC was packaged with N2 (Tables 1 and 2).
Table 1 Retention percentages of vitamins (%R-V) in CP+FAC packaged with atmospheric air and with N2 during storage at three temperatures (15, 25, 35 °C) and during 0, 30, 60, 90, 120, 150, and 180 days.
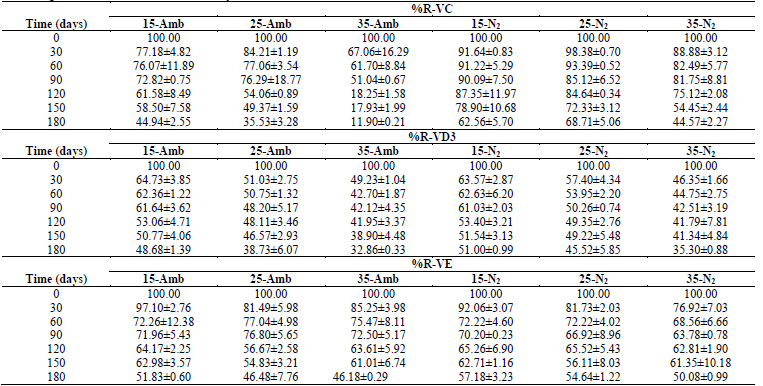
Source: The Authors.
Table 2 Retention percentages (%R-) of antioxidants in CP+FAC packaged with atmospheric air and with N2 during storage at three temperatures (15, 25, 35 °C) and 0, 30, 60, 90, 120, 150, and 180 days.
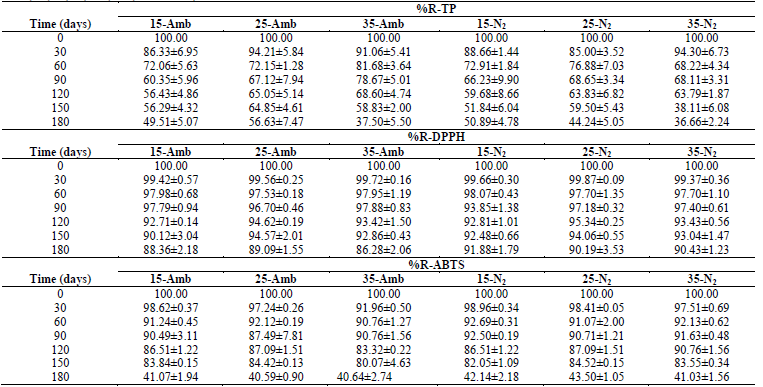
Source: The Authors
This losses are associated with multiple factors, related with storage conditions, like pH, TA, and tS, presence of catalysis of metal ions, concentration of salt, sugar, oxygen, enzymes, light, increased aw, as well as Xw, thermal treatment applied to the product (Table 1) [2,16,20,24,26-29].
Stability studies are limited and contradictory; degradation of VC, VD3, and VE during storage could be related to the reactivity with the oxygen present in the samples packaged with atmospheric air and to the presence of ferrous ions, which - in part - explains the greatest loss of VC when compared with that packaged with N2, where, by it being an inert, colorless, odorless, and insipid gas, it does not react chemically with other substances and has low solubility. It is used as oxygen substitute and displaces it in the packaging head space to avoid the development of aerobic microorganisms and oxidation problems [16,20,24].
Hymavathi and Khader, [30], Chávez-Servín et al., [4], Liu et al., [31] and Bosch et al., [32], reported significant losses of L-ascorbic acid, which increased with increased tS and TA in mango powder, powdered milk for infants, powdered tomato, and fruit-based foods for children, respectively; oxidation of L-ascorbic acid starts with the formation of dehydroascorbic acid, which can undergo a series of reactions with amino acids that lead to the active formation of pigments.
Udomkun et al., [1] and Prodyut et al., [33], with dry chunks of papaya and pineapple, respectively, reported that degradation of ascorbic acid (AA) accelerated exponentially during the first three months of storage in different types of packaging, which is associated with increased aw, as well as Xw, given that free water can act as a solvent for reagents and as a catalyst. Hemery et al., [34], reported losses of VD3 and VE during storage of fortified soy oil, when the oils were exposed to natural light; the factors with greatest influence on the retention of vitamin D3 were content of α-tocopherol and the interaction between time and type of oil.
Syamila et al., [16], found instability when evaluating the effect during storage of temperature, oxygen, and light on the degradation of β-carotene, lutein, and α-tocopherol in spray dried spinach juice powder, where the reduction of oxygen levels through vacuum packing and exclusion of light intensity below 800 lux did not improve the stability of the micronutrients. This made it possible for the residual oxygen in the powder to be sufficient to cause the degradation of the nutrients under study.
Romero-Braquehais [24], evaluated the behavior of VD and VE under storage in liquid milk at different temperatures (23 - 30 and 37 °C), demonstrating the effect of temperature on the instability of vitamin D, with 37 °C being where the highest loss occurs during the first three months of storage.
This behavior was similar to that found in the present work. It is known that vitamin D is susceptible to an alkaline pH range, light and heat, lipid oxidation, and to small amounts of transition metals.
Retention percentages of the antioxidant capacity %R-TP and %R-DPPH degraded linearly throughout the storage period, except for %R-ABTS that accelerated during the last 30 days of storage (Table 2), which is associated with TA, tS, pH, as well as with exposure to oxygen, light, changes in Xw, aw, RH, formation and accumulation of melanoidins, along with Maillard reaction products and type of packaging material, where the availability of oxygen for oxidation reactions as a result of the different gas permeability of the packaging materials, the oxidative enzymes, like polyphenoloxidase, or the chemical oxidation of phenolic compounds [1,2,7,14,28,29,35-37].
3.2. Degradation kinetics of the FAC
Losses of vitamins and of antioxidant capacity followed different kinetic models of degradation, where VC, DPPD, and ABTS had zero-order reactions, while TP were of first order and VD3 and VE of second order for both the CP+FAC packaged with atmospheric air and with N2 (Table 3).
Table 3 Kinetic degradation coefficients and statistical parameters of the regression analysis of the functionally active compounds of coconut powder under different storage conditions.
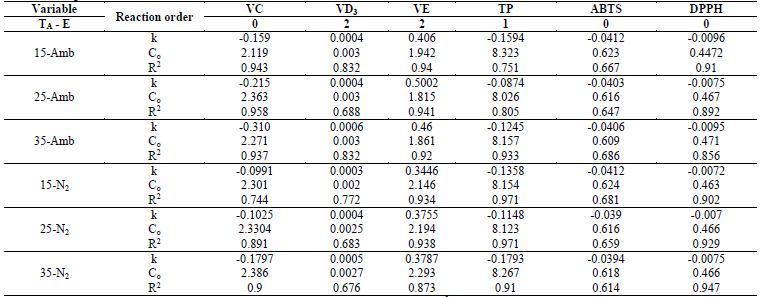
k: rate constant; -(-) the minus sign indicates FAC degradation; Co: Initial concentration; R2: determination coefficient.
Source: The Authors.
In VC, the correlation coefficients (R2), for all temperatures and types of packaging had - in general - values (0.9; the values of k show the degradation rate, which are higher for that packaged with ambient air than with N2 (higher values of k, lower stability) (Table 3). Similar behavior was reported by Romero-Braquehais [24], in liquid milk. While Shinwari and Rao [29], reported that ascorbic acid in berry marmalades and jams is more sensitive during storage after first-order degradation kinetics, where the storage temperature has a significant effect.
The effect of storage temperature on the degradation of VC determined via the Arrhenius equation, under zero- and first-order kinetic models, yielded that the activation energy (Ea) for the first-order kinetic model was 44.679 kJ/mol, and for the zero-order model it was 24.584 kJ/mol, in ambient packaging, while values of CP+FAC packaged with N2 were lower at 27.317 and 21.743 kJ/mol, for each kinetic model respectively; while the correlation coefficients (R2), were higher for the zero-order kinetic model, in both types of packaging (Ambient - 0.993; N2 - 0.978) (Table 4). Similar results were reported by Romero-Braquehais (2008) [24] in liquid milk regarding the zero-order kinetic model and R2= 0.99, but lower regarding Ea= 13.91 Kcal/mol. The difference in Ea with respect to those obtained in the different studies may be due to the different temperature ranges and to the different environmental conditions, such as oxygen, humidity, pH, and - of course - by the composition of the matrix [38].
Table 4 Influence of storage temperature and packaging type on A, Ea, and statistical parameters (R2) in degradation of functionally active compounds in coconut powder.
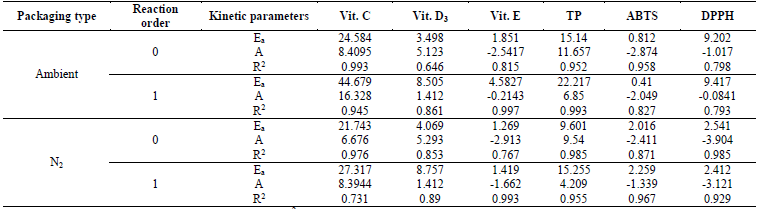
Ea: activation energy (kJ/mol); A: pre-exponential factor; R2: determination coefficient.
Source: The Authors.
The Ea can be expressed as the energy barrier the molecules need to cross to be able to react. The proportion of molecules capable of doing so increases with temperature; which is why the effect of temperature on the rates and the physical significance of A represents the rate constant at which all the molecules have enough energy to react (Ea= 0) [24]. The activation energy indicates, somehow, how fast the reaction rate is. In this case, the activation energy for the zero-order kinetics was inferior to that obtained by the first-order kinetics, indicating that VC degradation is higher in zero-order kinetics than in first-order kinetics. Romero-Braquehais [24], Bosch et al., [32], evaluated the degradation kinetics of ascorbic acid (AA) in liquid milk and in fruit-based foods for children during storage, reporting a first-order kinetics, where the values registered for k, obtained for the first-order kinetics were 0.05415, 0.2199, and 0.7469 at 25, 37, and 50 °C, respectively, thus, confirming the degradation of AA dependent on temperature and the value of the Ea was 20.11±0.33 kcal/mol, proving that the highest kinetic degradation of the FAC is obtained with lower Ea.
The degradation kinetics of VD3 had second-order reactions, where k values, which show the rate of degradation, were very similar, both for that ambient packaged and that packaged with N2 (Table 3). The correlation coefficients (R2), for all temperatures and types of packaging, generally had relatively valid values (0.67 and (0.84, values similar to those reported in liquid milk by Romero-Braquehais [24], showing first-order kinetics and with scarce data available in the literature, it is not possible to ensure if the losses of vitamin D follow a zero-, first-, or second-order kinetic equation, thereby, it would be necessary to increase the tS or the TA to have a clearer vision. Regarding the effect of temperature on VD3 degradation, the first-order kinetics presented R2 (0.86 for that packaged with atmospheric air (EA) and that packaged with N2 (EN2), while those of zero order had R2 = 0.646 and 0.853, respectively. The Ea for the zero-order reaction were 3.498 (EA) and 4.069 (EN2) kJ/mol, lower than those of first order at 8.505 (EA) and 8.757 (EN2) kJ/mol, suggesting higher dependence on temperature in the rate of VD3 degradation (Table 4).
Vitamin E had a second-order kinetic degradation model, for all temperatures and packaging conditions, with similar behavior for liposoluble vitamins, the R2 were (0.9; rate constants (k) were higher for the samples packaged with atmospheric air than with N2 (Table 3), which indicates a slower degradation of the VE packaged with N2. The effect of storage temperature on the degradation of VE, for the zero-order and first-order kinetics, showed R2 (0.75 and (0.99 for EA and EN2, respectively, while the Ea was lower for the zero-order reactions and for that packaged with N2 (Table 4).
Romero-Braquehais [24], reported for liquid milk first-order degradation kinetics of VE, and Ea similar to those found in this work. Syamila et al., [16], in powdered spinach, reported first-order kinetics for α-tocopherol, showing a gradual decrease of the content through the storage time. The high rate of degradation of nutrients with low water activity suggests that the reaction was of self-oxidative nature, and it could be argued it was due to the presence of residual oxygen in the packaging. It is known that the dissolved residual oxygen is responsible for the deterioration of β-carotene and α-tocopherol during long periods of storage, even if the packaging head space is purged with N2.
With respect to the degradation of antioxidants; TP had first-order degradation kinetics for all temperatures and packaging conditions, where k were higher for EA (more susceptible to degradation), but the higher R2 for EN2 ((0.9) (Table 3). The effect of storage temperature on TP degradation determined through the Arrhenius equation, under the zero-order and first-order kinetic models, yielded that the activation energy for the first-order kinetic model and for the EA, was higher than for those of zero order and EN2 (Table 4), and the R2 were (0.95. The aforementioned agrees with that reported by Shinwari and Rao [29], where the TP diminish during storage in jams and marmalades at high storage temperatures, bearing in mind the composition; where pectin stabilizes highly reactive compounds, like anthocyanins, vitamins, and antioxidants through hydrogen or the hydrophobic union. The magnitude of these interactions is based on the type and concentration of pectin. Where pectins with a low degree of esterification minimize the loss of bioactive compounds and retention is better in higher concentrations of pectin. Likewise, the stability of the functional compounds varies with the type and variety of the fruit, given that they differ in their composition (sugar, pectin, minerals, etc.).
The loss of antioxidant capacity, determined for ABTS and DPPH, presented zero-order degradation kinetics, with the same behavior in the values of k and R2, although in ABTS, the values of R2 ranged between 0.61 and 0.69; while DPPH (0.9; better describing the behavior of loss of antioxidant capacity in these (Table 3). While the Ea and R2 of the effect of the temperatures under the zero-order and first-order kinetic models in the DPPH were higher for the EA, but the R2 was lower than the EN2, presenting the contrary effect in ABTS in the Ea, but similar R2 and (0.82 (Table 4).
Kim et al., [39], reported that the degradation of the total phenolic content and the antioxidant activity (DPPH) in kiwi (Actinidia arguta) puré, stored at various temperatures between 5 and 45 °C during 72 h, followed a first-order kinetic model and indicated a strong dependence on the temperature of the phenol content and the antioxidant activity, thus, the degradation of the phenolic compounds and the antioxidant activity occurs faster with increased temperature and storage time, with values of Ea of 28.15 and 29.07 kJ/mol, respectively.
Zorić et al., [14], studied the phenolic degradation kinetics of cherry powders and found a first-order reaction, represented by a more progressive loss at higher storage temperatures and it was confirmed by kinetic parameters, where the rate constant (k) varied between 0.02 and 0.067 and the activation energy (Ea) between 10.956 and 31.59 kJ/mol and the R2 were between 0.64 and 0.99, finding that the highest phenolic degradation in powders takes place with lower Ea; a similar behavior was found in the present work.
Henríquez et al., [35], with apple peel powder and using two packaging materials (high-density polyethylene, HDPE, and metalized high-barrier films, MHBF), observed that phenols diminished during the storage period in both packaging materials tested, independent of the storage conditions (temperature and RH) used. This was confirmed by the kinetic parameters of the phenolic degradation throughout the storage time, where in all cases, the regression analysis suggests a zero-order kinetics, with a relatively lower value of Ea in HDPE compared with MHBF (3.038±84.85 cal/mol K and 3.220±157.03 cal/mol K, respectively) and where the highest k values (constants for phenolic degradation) in the first package could suggest that the degradation of phenols may be associated with the product’s humidity gain. Daza et al., [37], with cagaita (Eugenia dysenterica) powder, reported that degradation of phenols exhibited first-order kinetic behavior. In effect, the loss of attributes of food quality takes place in higher humidity conditions, when there is greater mobility of the reagents and dissolution of organic acids.
Udomkun et al., [1], in dry papaya, evaluated the effects through type of packaging (aluminum and polyethylene films, ALP, and polyamide/polyethylene, PA/PE, films) and through tS over the antioxidant capacity and the total phenol content, reporting that the quality parameters followed zero-order reactions, except for ascorbic acid (AA), which was described better by using a first-order quality model parameter and where the R2 were >0.95; and the values of k, which show that the rate changes were more drastic for the samples stored in PA/PE packages compared with those in ALP bags, meaning that greater degradation was produced in dry papaya when packaged in PA/PE.
4. Conclusion
The degradation kinetics of the FAC during storage, under the conditions described, followed different kinetic models of degradation, where VC, DPPD, and ABTS had zero-order reactions, the TP were of first order and VD3 and VE of second order; being higher with %R-Vitamins and Antioxidants at lower TA, and in the treatments where the CP+FAC was packaged with N2. At the same time, it was possible to determine the strong dependence on temperature of the reaction constants, which was explained by the Arrhenius ratio, where increased storage temperature reduces FAC stability and it was possible to determine that the greatest kinetic degradation of the FAC is obtained with lower Ea.