1. Introduction
Formation damage is a general term that refers to the reduction of oil and gas wells injectivity and productivity through several adverse mechanisms [1-3]. Consequently, formation damage stands as an operational and economic undesirable problem that could happen during different exploitation phases, including drilling, production, hydraulic fracturing, and workover operations [1,4,5]. Many factors could trigger formation damage, including physicochemical, chemical, biologic, hydrodynamic, and thermal interactions between the porous medium, reservoir fluids and/or injection fluids. Further, different types of formation damages can co-exist in the same reservoir. Formation damage mechanisms are generally divided into that related near-wellbore or improved oil recovery operations (IOR) and those characteristic of EOR methods. Fig. 1 summarizes the formation damage mechanisms in each situation.
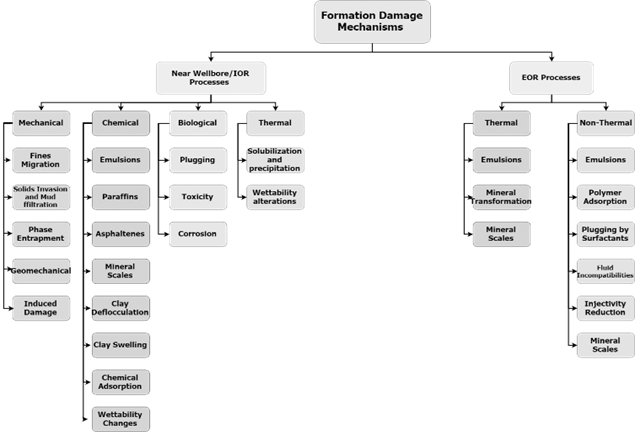
Source: The Authors.
Figure 1 Main mechanisms of formation damages generated in near-wellbore/IOR operations and EOR processes.
In the case of near-wellbore/IOR, these can be divided onto mechanical, chemical, biological, and thermal mechanisms. In the case of EOR methods, these are classified according to the thermal or nonthermal method employed. Within the types of formation damage mentioned, depending on the proximity to the well, damage associated with the flow regime in the reservoir can occur when there is spherical or hemispheric flow, and after radial flow during linear, dual linear and parabolic flow regimes. All the mentioned formation damages can change with time, increasing their magnitude and decreasing the production of wells, mainly in gas wells [6,7]. Formation damage is not necessarily reversible [8,9] and its inhibition is a better option than its remediation as some consequences are the reduction of oil and gas production and recovery, higher energy consumption, decrease in the lifespan of the well and higher production costs [8,10]. Further, different experimental, modeling, and simulation techniques adequately designed allow the comprehension, diagnosis, and prevention of formation damage in oil and gas fields [11]. Laboratory experiments are essential for achieving full knowledge of the different formation damage mechanisms [12]. From this experimental framework, models that allow extrapolation to field conditions can be useful [11,13]. These efforts are necessary to develop and verify precise mathematical models and simulators that can be used to predict and determine strategies to avoid and mitigate formation damage in oil reservoirs [12,14].
In this sense, the planning and design of field test procedures from the use of mathematical models are vital [12,15]. Once a model has been validated, it can be used to simulate with great precision the formation damage and the effectiveness of treatments for its inhibition and remediation. However, current techniques for reservoir characterization by historical comparison do not consider changes in the formation properties through the productive life of the reservoir
Hence, models that can characterize the variations of these variables accurately through time using a history match are necessary to predict future performance [16-18] precisely. Thereby, the methodology for diagnosis, quantification, disaggregation, and characterization of formation damage processes allows the engineer to formulate an effective treatment that achieves to prevent, remove, and inhibit the damage mechanisms of greater relevance that are affecting the productivity [16]. In this sense, it is necessary to consider the monetary factor in terms of the treatments implemented in oil fields in the past [17]. Nevertheless, remediation operations without a conscientious design could lead to additional formation damage mechanisms [18]. Hence, different authors have developed methodologies that allow determining and quantify the impact of each of the sources of damage, ensuring successful and cost-effective remediation and inhibition interventions. This document presents a review of different disaggregation and discretization methods for formation damage estimation in oil and gas fields. The first section shows the current main strategies for formation damage diagnosis. The second section presents the review of different mechanisms for diagnosis and quantification of formation damage. Finally, the third section accounts for the description of different formation damage disaggregation methodologies.
2. Formation damage diagnosis
To identify the possible causes of production decay in one or several wells, a multidisciplinary analysis is necessary since there are too many factors that could contribute to this situation, e.g., an inefficient mechanical state, low permeability, low reservoir pressure, damage, and pseudo-damage, or by natural causes. Hayatdavoud et al. [19] recognize that such factors are of prime importance as they help to understand a premature oil production drop, allowing taking preventive-corrective measurements before the appearance of severe damage.
Yeager et al. [20] indicated that there is not a single tool such that the only necessary and important information could be obtained from it. Hence, it is required to perform several diagnosis tests, among which are pressure test analysis to determine if there is any damage quantitatively, well bottom record to see any damage on formation areas, well bottom liquid and solids sampling and biopsy-like rotatory core sample from wellbore lateral face in open hole completions. Also, Yeager et al [20] endorse a well bottom video before other diagnostic tests and sampling to evaluate the presence, nature, and morphology of the wellbore surface and perforations deposits.
2.1. Well testing
Well Testing through pressure tests gives information about the relationship between formation capacity or permeability thickness (i.e., permeability multiplied by formation thickness) and formation damage skin factor defined as “S”, which is one of the most important and representative variable for reservoir characterization test. However, this technique often requires large amounts of dead times as well as additional PVT information. Also, well testing techniques are megascopic techniques that will yield results in the megascopic realm. Therefore, microscopic and macroscopic techniques are also needed to be coupled with well-testing results to comprehend and validate the data fully. Nonetheless, one method is not enough to perform a proper analysis of wellbore and reservoir properties, even more, when dealing with different formation damage mechanisms. Nevertheless, these tests only give information at the moment they are taken, so an effective formation damage evaluation must be done through several pressure tests. Considering corrections by non-Darcy flow in high turbulence producing wells and inertial effects advocates an approach based on three steps that consist in quantify existing formation damage, diagnose the different formation damage mechanisms and perform laboratory studies to increase current knowledge on specific mechanisms [21].
2.2. Build-Up pressure tests
Build-Up pressure tests are performed by closing a wellhead Christmas tree on wells that were operating at a constant known flow rate and then measuring the pressure building or accumulation during the shutdown period [21]. The accumulation time required to reach reservoir pressure during the shutdown period depends on the permeability [21]. Therefore, a Build-Up pressure test could give an early indicative of formation damage presence, as when the pressure is restored quickly during a shutdown period while a considerable difference between the initial and final flow pressure in a short period exits. This means that a pressure transmission exists with a low flow transmission probably associated with a permeability obstruction. This test does not require supervision and could potentially give an estimation of the initial reservoir pressure if the well has not been producing for too long. Nevertheless, this test requires the well to be closed and this might not be economically appealing. Now, to show how the phenomena are viewed, consider a well that produces at a constant flow rate from an infinite, homogenous and isotropic reservoir that involves a radial cylindric flow regime, denominated fundamental flow unit [21]. The accumulation shutdown pressure behavior during the transition period was described by Horner et al. [22] expressed by the Eq. 1:
where, P i is the initial reservoir pressure, t p is the shutdown period time, Δt is the time elapsed since the well’s shutdown, q is the production constant flow rate before the shutdown.
Using the given slope in Horner’s plot, permeability thickness K h and skin formation damage factor S could be determined using the given equation [22]:
2.3. Draw-Down flow test
The well is closed long enough to reach static formation pressure [23]. Once it is reached, the well is opened to flow at a constant rate while bottom-hole flowing pressure is measured through time. Despite being less used as PBU’s tests, flow tests do have some advantages over the later. First, unless it is a decline test, the well is not required to be closed during the run, so production is not stopped. Also, it is not necessary to re-start production, however in this type of test, supervision is crucial, and information about S and K parameters could not be representative.
2.4. Tiab’s direct synthesis technique
A log-log plot of pressure and pressure derivative versus test time may reveal the presence of several straight lines corresponding to different flow regimes; bilinear flow, linear flow, infinite-acting radial flow, and pseudo-steady state flow. The slopes and points of intersection of these straight lines are unique and, therefore, can be used to calculate several well and reservoir parameters such as permeability, skin factor, wellbore storage coefficient, and drainage area [24,25].
2.5. Production history analysis
The production history of a well should contain oil and water production details, water cuts, oil gas relationships, changes in the production system, decline curves, among others [26]. For example, if the artificial lift system is working inefficiently, a high fluid level should be observed, or high bottom-hole pressure, added to low production rates, or an abrupt production fall not associated to workover operations could be analyzed based on two main criteria: if it is related to sudden water production, then it could an indicator of migration and accumulation of fines in the critical near-wellbore area or if it is not, then it could be associated with asphaltene deposition near-wellbore face. On the other hand, a smooth decline in production could be related to a gradual accumulation of paraffin deposits in the pipeline. If any formation water incompatibility is encountered in injection processes of secondary recovery and a drop on oil production is perceived, it could be associated with mineral scale deposition.
3. Formation damage quantification
Formation damage could be quantified based on several factors, such as formation damage skin factor, changes in relative permeability, fluid viscosity, fluid mobility, flow rate, flow efficiency, and formation damage depth.
3.1. Skin factor estimation from well testing
Pressure tests are tools for both diagnosis and quantification of formation damage whenever they are available. Earlier, the procedure to determine formation damage skin factor by both Build-Up and Draw-Down well testing was described. Nevertheless, well testing is not the only tool available. Through Drill Stem Testing, an approximation to formation damage caused by solids and mud filtrate invasion could also be determined on the wellbore face, identified by a reduction of the invaded zone permeability. The following equation models the change in pressure as a function of time for this test:
where u* is the maximum square root greater than 1/2.
A plot of P D vs. t on semi-log scale corresponds to two straight-line represented by early and late time regions in which the first region is affected by formation damage, from which K could be obtained. Late time region is not affected by formation damage, so from this straight-line K could be computed.
3.2. Type curves
Type curves are interpretation curves extracted from several Build-Up or Draw-Down pressure tests, in which a storage and formation damage factors are considered as internal boundary conditions for the diffusivity equation solution. Type curves are plotted on a log-log scale, where the ordinate is represented by the pressure drop during the test, and the abscissa is represented by time or a function of this depending on the type of well test performed.
Diffusivity equation solution for the internal boundary storage condition and formation damage is given by the Laplace transform domain by:
The inverse of Eq. (6) is a function of dimensionless time, formation factor S, and the storage parameter C
sD
. This solution is represented in a graphic form as mentioned earlier with S and C
sD
as parameters. In the ordinate P
D
is plotted, and in the abscissa t
D
, the interpretation of pressure tests based on type curves relies on the fact that a log-log P
D
vs. t
D
plot has the same behavior as a log-log ΔP vs. Δt plot plus a factor that corresponds to the pressure and time constants for each case respectively. The second plot is built from restoration or flow pressure test data so that both plots coincide with documented type curves found on literature. When this happens, Storage and skin parameters are found. When it comes from a flow test, the pressure difference is defined as ΔP = P
i
-P
wf
and Δt is the test duration. For a Build-Up pressure test, these parameters are defined as
and
, where t
P
is the production time before the test begins, Δt is the period in which the well has been closed, P
w2
is the pressure recorded during a lapsed time Δt since it was closed and P
wf, Δt-0
is the instantaneous pressure at the shutdown time.
Some examples of type curves are Ramey: P
D
vs. t
D
, whose parameters are: S and C
sD
; Gringarten: P
D
vs t
D
/ C
sD
, whose parameter is: C
sD
e2x
; Bourdet:
vs. t
D
/C
sD
which makes Gringarten interpretation easier, and McKinley that does not provide an estimative for skin value.
3.3. Multi-parameter model
The multi-parameter model for the skin factor characterization was proposed by Restrepo et al. [16]. It is a methodology of formation damage study based on statistical correlations that allow the classification of different damage mechanisms present in a well [16,27]. The model involves six types of damage: Mineral Scale, Organic Scale, Fines Blockage, Induced Damage, Relative Permeability Parameter, and Geomechanical Damage. Initially, the method proposes to study each kind of damage separately and the critical variables for each phenomenon.
For this, saturation pressure, production rates, petrophysical, and fluid properties should be entered. Moreover, for a set of wells with similar characteristics to the studied well, data related to damage parameters such as colloidal instability index for asphaltenes, mineral scale index, critical flow rates, and filtration rates are required. In this sense, the parameters associated with the damage mechanisms mentioned are: Mineral scaling parameter (MSP), Organic Scaling Parameter (OSP), Fines Blockage Parameter (FBP), Induced Damage Parameter (IDP) and Relative Permeability Parameter (KrP). MSP parameter is calculated with four subparameters: Index of CaCO3, of BaSO4, of iron scales, and calcium concentration on back flowed samples. OSP parameter is calculated through the calculation of 4 sub-parameters that are: CII factor, chemical alteration factor, and compositional factor.
Besides, the FBP parameter is calculated through the calculation of 5 sub-parameters that are: Aluminum, and silicon concentration on produced water, critical radius factor, mineralogical factor, and crushed proppant factor. By contrast, IDP parameter is calculated through the calculation of 3 sub-parameters that are: Mud damage factor, polymer damage factor, and invasion fluids factor. Finally, KrP parameter is calculated through the calculation of 3 sub-parameters that are: reservoir pressure, delta pressure prom saturation pressure and water intrusion factor. The statistical analysis takes available data from all wells and generates a cumulative frequency distribution of each variable per damage mechanism, allowing to know the ten percentile (P10) and the ninety percentile (P90) of different profiles. Restrepo et al. [16] proposes, also, a normalization procedure of damage variables to calculate the level of influence of these parameters over a particular mechanism. The normalized values are plotted on a spider graph in which the corners represent the critical variables for a specific type of damage [16].
Next, relative statistical weight is estimated for each type of damage as the average of its normalized critical variables. The authors suggest that depending on the formation damage mechanism, the affected radius will be deferent. This damage radius r d is obtained considering the critical pressure that leads to the phenomenon (e.g., onset pressure in the case of asphaltenes) along with the pressure profile of the well. As a final result, the multi-parameter model allows knowing the equivalent skin factor for each damage mechanism and their contribution to the global damage by Hawkins equation [28]. A commonly used illustrative way to represent the results of this methodology is by using a spider graph, as the one shown next in Fig. 2 [29]:
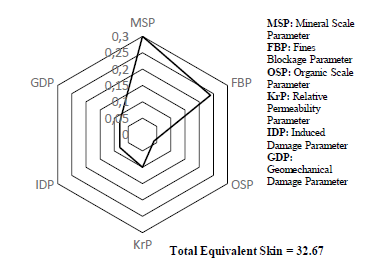
Source: Adapted with permission from Suárez et al. [29]
Figure 2 Formation damage percental representation in a spider graph. For the specific case represented by the spider graph, a high percentage of damage by mineral scales is observed approximately 30%, followed by a fines migration damage with 20%, and with a lower degree of condensate damage, induced, Kr and organic.
3.4. Formation damage quantification indexes
Using pressure and laboratory tests, it is possible to determine properties that are affected by formation damage such as permeability, viscosity, mobility, among others. Several indexes could represent the formation damage of these properties. Some of them are presented below:
3.4.1. Relative change in permeability, (RCP)
where K d is the damaged permeability and K is the original formation permeability [8].
3.4.2. Relative change in viscosity, (RCV)
The RVC represents the change in fluid viscosity by any type of formation damage mechanism, i.e. emulsification.
Where µ and µ d are the fluid viscosity before and after formation damage happened [8].
3.4.2. Relative Change in Fluid Effective Mobility, RCEM
Effective mobility of a fluid is a measure of its capacity to flow across the porous media, and it is defined as:
It has been observed that asphaltenes presence causes crude oil mobility to decrease through many mechanisms, whereby an index is proposed in the following equation:
Where λ and λ d are fluid mobility before and after formation damage occurs [8].
3.4.3. Relative change in flow rate (RCFR or DR)
With flow equations for q and q d proposed by Amaefule et al. [30] Craft et al. [31], DR index is described below:
Amaefule et al. [30] proposed a way to estimate the economic impact formation damaged caused as a function of annual economic loosed profit (FD$L) [30]:
Where P is the oil price at a specific time and q is the flow rate of produced oil at the same particular point of time.
As mentioned earlier, production decline could also be associated with alterations in reservoir fluids characteristics. Emulsification could be one of such mechanisms in which oil and water phases mix up together to form an emulsion with much higher viscosity due to an increase in flow velocity near the wellbore area where the pressure gradient is more significant. Both direct and inverse types of emulsions increase viscosity. However, water in oil emulsion causes a more considerable rise in viscosity that could be several magnitudes above the original system viscosity. This type of emulsions creates a permeability blockage that obstructs fluid movement. For this case, theoretical and real flow rates are defined, and a “viscous skin effect" DR expression is defined as follows:
where the subscript d represents block emulsion properties and r w , r d and r e represents wellbore, damaged zone, and reservoir radius, respectively. For this, the authors proposed an expression for skin effect, as shown next [8]:
3.4.4. Flow efficiency
FE is the relationship between flow efficiency (FI) of the damaged and non-damaged formation:
where
and P
wf
represents average reservoir pressure and bottom-hole flowing pressure, ΔP
s
is the additional pressure drop near-wellbore as a result of formation damage [32]:
3.5. Formation damage disaggregation
Civan et al. [12,14] proposed a general formation damage model to simulate on a broad spectrum of formation damage processes using different schemes proposed on literature. To identify dominant formation damage mechanisms and their contribution to the system, five key processes are defined. These are chemical, physic-chemical, hydrodynamic, thermal, and mechanical processes. The same authors propose that all modeling schemes have a common ground and as a result, disaggregates total formation damage as the individual contributions of each mechanism in the following way:
where i represent the different formation damage processes, S t is the skin damage factor associated with i, and S t is the total skin damage factor. Some of the methodologies described below, work on this hypothesis.
Few methodologies are currently reported for formation damage disaggregation. One approximation is based on the numerical pressure test data interpretation using simulators coupled with geomechanical models how has been done by López et al. [33] where total formation damage is disintegrated by drilling and completion damage component and a pressure depended on damage component. At the same time, this last component can be divided into thermodynamic damage (asphaltenes, inorganic scales, fines) and geomechanical damage.
Where S t represents total formation damage, P D is the dimensionless pressure, γ D is the dimensionless permeability module, S M represents mechanical damage, and SΔ k represents the damage caused by a reduction in permeability due to changes in pore pressure.
This method comes in handy to diagnose general mechanical-type damages. Still, when thermodynamic damages are more significant, this analysis does not give enough information as it does not study them in-depth. Another shortcoming it is that requires recent pressure test data, and the numerical interpretation implies an iterative solution of multiphase flow equations coupled with geomechanical analysis demanding a high computational cost using algorithm optimization.
Additionally, López et al. [33] developed a methodology for formation damage disaggregation in three main components: non-Darcy flow, geomechanical and mechanical damage through Build-Up pressure tests for gas reservoirs in which high flow rate causes turbulent flow. Using Spivey’s Model, the first division of formation damage is made into the non-Darcy flow, and another component called prime damage. Now, using the information of pressure tests for the well in combination with an ATS software for disaggregation, formation damage is characterized near the wellbore in non-Darcy flow and geomechanical damage. Combining these two steps, this methodology achieves to divide formation damage in three main contributions that are: non-Darcy flow, geomechanical, and mechanical damage.
Non-Darcy flow can be characterized through several methods, among these are modeling of turbulent flow through the following equation [34]:
Others methods are based on the calculation of non-Darcy flow through correlations [35], core analysis [36], and log-log type curves diagnosis from which an estimative of the Darcy and non-Darcy flow components through the use of Build-Up pressure test can be obtained using the following equation [37]:
Where P sf is the wellbore face pressure, P w is the wellbore pressure, S is the skin damage factor by Darcy flow, D D is the factor associated with non-Darcy flow, and q sfD fluid flow through the wellbore face. P wD dimensionless pressure could be found as:
Through an iterative process, when P wD satisfies tolerance criteria, Darcy and non-Darcy flow damage factors could be obtained. Finally, using Pedrosa’s equation [38] permeability change could be calculated:
where K i is the permeability at a reference pressure and γ D is the dimensionless permeability module defined as:
In an analogous manner to the earlier procedure, P wD the pressure is calculated and compared to the real value, and once the tolerance criteria are met, the iterative process will be completed, and mechanical formation damage will be obtained as a result. Finally, and using Civan hypothesis [12], as explained earlier, formation damage geomechanical factors associated with pressure changes can be calculated using Eq. 29.
Where S T is the total formation damage from Darcy (S D ) and non-Darcy (S ND ) damage factors contributions, S M is the formation of mechanical damage factor and S G is the damage factor by geomechanical damage.
On the other hand, Santamaria et al. [39] proposed a numerical methodology to diagnose formation damage in the function of flow rate, rock stresses, and overall damage, allowing to know which portion could potentially be remediated through chemical stimulation. This methodology is based on a mathematical algorithm that uses fundamental equations in addition to correlations developed on conceptual analysis and statistical experimental results from the petrophysics test. Santamaria et al. [39] propose an alternative methodology for the analysis of formation damage components starting from the phenomena involved in damage processes that use concepts such as hydraulic units, stress sensibility, and turbulent flow linked together to produce a proper conceptual model to work from. This methodology consists on determining which wells are better candidates to chemical stimulation considering the total formation damage as the summation of different contributions, and by a statistical and analytical treatment that allows identifying trends, variables correlations and adjustment degree. To achieve this, Santamaria et al. [39] analyze damage from two perspectives. The first characterized by changes in permeability, in which damage is of mechanical or physic-chemical nature, and the second one is focused on damage provoked by stresses or Non-Darcy flow. In this manner, formation damage its divided by factor and analyzed in terms of permeability module (damage by effective stress) and friction coefficient (damage by non-Darcy flow) thus, optimizing available information and treatment proposal [39]. As explained, Restrepo, et al. [16] developed a multiparameter model that allows determining the relative weight of each formation damage mechanism present in a well to implement proper corrective actions based on the most important mechanisms. However, it does not take into account the long-term behavior of the different formation damage mechanisms or the performance of treatments as well as economic return in the future, rather it gives a picture of a moment. In this regard, Suárez et al. [29] developed a hybrid model that consists of the multiparameter model, linked with a multiphase 3D simulator that studies the evolution of treatment performance on the well to evaluate different scenarios. Therefore, a tool that gives a more complete landscape in formation damage treatment implementation and economic return, since their model can run different scenarios in which several treatments and intervention schemes could be used so the financial return could be maximized [29].
Recently, a tool for formation damage management had been developed [40]. With this, it is possible to obtain through analytical and statistical damage characterization and discretization, well surveillance and accurate analysis of {formation damage in real-time to make better and earlier intervention operations based on the Integrated Formation Damage Model (IFDM). Besides, this tool provides an ever-increasing database which ultimately enhances well management. This tool was developed by Restrepo et al. [40] and uses an interactive interface build in Laravel, which makes it portable through internet access [40].Fig 3 shows the multiparametric module outputs for skin characterization in which the total damage has been disaggregated in different types of reservoir damages, including each contribution in a spider plot (see Fig. 3).
Techniques and treatment of damage remediation are expensive, and their formulation might be complex due to the different mechanisms that occur at the same time. This could lead to non-optimized treatment formulations. In this sense, it is introduced the concept of multivariable statistical analysis by which, through correlations matrixes, the most critical variables are identified to formulate a successful treatment, and by multiple regressions, possible results can be predicted. This methodology comes in handy in wells damaged by multiple types of damage, because of the statistical treatment that can identify the most significative variables, and based on this, formulate treatment in the most effective way [41].
Renpu et al. [42] advocate that the damage skin factor obtained from well tests is a parameter that not only refers to formation damage but also involves other elements from the drilling and completion phases that give additional pressure losses. Eq. (30) proposed by Rempu et al. [42] divides total skin discretization, S t , into skin generated by thermodynamically damage S d , and pseudo-skins composed by well deviation S e , non-Darcy flow S αD partial perforations penetration S P , well form factor S A , number of perforates S pF and perforations geometry S dp [42].
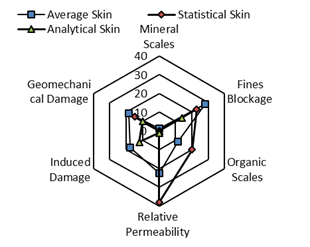
Source: With the permission of Restrepo et al. [40].
Figure 3 Multiparametric module outputs results for skin characterization.
To calculate each pseudo-damage, the author [42] propose a mathematical procedure that involves well deviation angle, horizontal permeability, depth, and perforations thickness, among others. The method contemplates the study of the damage concerning mechanical developments in the well. However, it does not specify the phenomena of formation damage caused by thermodynamic effects such as pressure, temperature, and stress changes.On the other hand, expert systems have been developed to simulate complex decision making fed by multivariate systems and expert opinions [43]. In this sense, Xiong et al. [44] developed a computational model, designed for gas storage wells, to disaggregate formation damage and select the best stimulation treatment. The model combines expert systems, neural networks, and algorithms together with knowledge, experience, results of numerous well tests, technical literature, and historical cases to identify the damage mechanisms present in a well. The qualities of expert systems give the methodology the ability to store data, learn from experience, make logical decisions and explain why, while simulating the reasoning of a specialist in the subject. In this order, the system was developed in two steps: knowledge acquisition and knowledge representation. In the first one, all possible data were obtained from multiple experts, in addition to actual operators, so the knowledge system base could continuously be updated. Then, the second step involves data structuring in which the required knowledge was divided into four main categories: candidate selection, damage mechanism diagnosis, treatment recommendation, and treatment evaluation. As a result of the model, the possible types of damage that are presented in the well are obtained based on input data, categorized from higher to lesser importance, select the possible treatment, fluids, and additive for intervention that could be used by any engineer with ease. Even so, it has the disadvantage of not considering the damage caused by geo-mechanical phenomena, being designed only for gas storage wells, and relying on excessive information and input data [44].
On the other hand, Garrouch et al. [45] develop an expert system for the identification and quantification of formation damage by clay swelling, fines migration and deposition of organic and inorganic scales divided into two modules, one for clay swelling, deposition of inorganic and organic scales and another for quantification of the sludge formed by asphaltenes based on empirical, technical knowledge and results found in the literature. Specifically, asphaltene precipitation module can estimate the precipitation potential by the rule of thumbs criteria based on the type of crude oil and solvent used for a broad range of different types of reservoir fluids. In the other hand, the amount of sludge that asphaltenes could form is calculated based on the crude oil acidity, surfactants as well as other additives. The results presented for the modules of asphaltene precipitation and sludge formation indicate that asphaltene precipitation is important in the case of light crudes with high content C 1 -C 3 and low content of C 7+ fraction. Regarding the formation of sludge, the expert system finds that concentrations higher than 15% of HCl severely increase the tendency to form a slurry, in the same way, concentrations greater than 3% of hydrofluoric acid (HF) aggravate the damage by sludge formation [45].
4. Conclusions
The process of assessing the formation damage is multidisciplinary, a consequence of the complexity that this phenomenon represents. From start to finish, all stages must play a complementary role. Because of this, it was determined that a remediation scheme of formation damage should contemplate roughly all steps of diagnosis, quantification, disaggregation, and characterization, as each of this step are just as important on its own to the selection program of the remediation technique that ensures the maximum collateral damage reduction and the successful fulfillment of all objectives This article is a complete guide of all the factors and processes to be considered in the search to avoid, reduce, characterize, disaggregate and quantify the formation damage.