1. Introduction
Metal oxides such as iron, cobalt, copper and zinc are candidates for a variety of important technological applications in catalysis, solar energy conversion, electronics, magnetic media storage, etc. [1]. Recently, nanomaterials based on such oxides have been developed for various applications. Among these nanomaterials, Co3O4 cobalt oxide has several morphologies and advantages, such as biological compatibility, broadband, high stability and low cost [2]. Due to its good reproducibility and selectivity, this material has also been explored in fields such as supercapacitors and electrochemical sensors [3-5].
It is known that the behavior of nanophase materials depends largely on the shapes and sizes of the particles, which are, therefore, key factors in their performance and final applications [6]. Different electrical, optical, magnetic and mechanical properties in semiconductors can be achieved with a controlled size of nanoparticles. Nanostructured porous materials also have applications such as gas sensors, catalysis, among others [7,8]. Transition metal oxides with various oxidation states are very promising candidates for the high capacitance of the next generation, including nickel oxide. Regarding nickel, it has shown a very high specific capacity, low cost, low toxicity and respect for the environment, but with a relatively low cyclic reversibility [9].
The synthesis of cobalt oxide compounds with adjustable physicochemical properties has become a research point, where doping with transition metals improves properties such as electrical behavior as well as optical absorption [10]. Specifically, doping of Co3O4 with nickel has shown significant changes in the behavior of the material. Improvement in the catalytic activity for the oxidation of CO through hybrid matrices is found in the most recent works of nickel doped Co3O4 [11]. Wang et al. [9] evaluated the performance of solar-powered steam as a possible candidate for solar photothermal conversion using Co3O4 nanoforest / Ni foam. Li et al. [12] analyzed the ORR activity according to the influence of nickel doping content. Ouyang et al. [13] concluded the excellent capacitive performance of the composite material attributed to the improved charge transfer rate and ion diffusion path. Additionally, Ren et al. [14] indicated that doping with Ni in the spinel lattice of Co3O4 improves reaction kinetics and promotes catalytic activity.
In the present research, Co3O4 and Co3O4 doped with 4% nickel were synthesized by the hydrothermal technique and then calcining them at 300°C. The products obtained will be characterized using different techniques, such as X-ray diffraction and subsequently Rietveld refinement analysis. Samples of Co3O4 have a crystalline structure after final heat treatment, and secondary phases belonging to the precursors used in the synthesis. SEM was used to observe the particle size and band gap results were obtained by UV-Vis. The research seeks to establish a comparison between Co3O4 and Co3-xNixO4 x=0.04 studying the effect of hydrothermal synthesis.
2. Materials and methodology
2.1. Preparation of Co3O4
The synthesis was performed following the procedure of Jin et al. [15]. Initially, cobalt acetate and distilled water were used in the synthesis, then, urea and ammonia were added with constant stirring. The solution was carried in an autoclave for 20 hours at a temperature of 180 ° C. Finally, the sample was washed with distilled water twice and the powders obtained were calcined at 300 ° C for 3h.
2.2. Preparation Co3O4 / Ni (4%)
Cobalt acetate was dissolved in distilled water. After solubilizing the components, nickel sulfate and, subsequently, urea were added. 2ml of ammonia were also added to adjust the pH. The solution was transferred to an autoclave and heated for 20 hours at 180°C. The samples obtained were washed with distilled water and ethanol and dried for 7 hours at 60°C. Finally, the powders were calcined at 300°C for 3 hours. Fig. 1 shows the schematic diagram of the hydrothermal process for the synthesis of nickel-doped Co3O4.
2.3. Characterization
The characterization of the samples was carried out using the techniques described below. The X-ray diffraction analysis (XRD) was performed on a PANalytical X’pert Pro equipment with data taken at an angle 2 between 100 and 800. It uses a RTMS (real time multiple strip) detector with CuKα radiation, a wavelength of λ = 1.542Å and a step of 0.02630 in Brentano Bragg mode, with subsequent Rietveld refinement of the experimental data, using the GSAS II code.
A Cary5000 UV-Vis-NIR diffractometer was used for measuring the UV-Vis reflectance spectrum in diffuse reflectance mode in the 200-2500 nm wavelength range. With the aim to study the optical response of the Co3O4, the samples were measured with UV-visible electronic absorption spectroscopy. The morphological analysis was performed with a FEI Quanta 200 scanning electron microscope (SEM), in secondary electron mode at high vacuum and voltage of 30 KV. The qualitative chemical composition analysis was performed via energy dispersion microscopy (EDS).
3. Results and discussion
3.1. Structural characterization
The spinel structure tolerates a high concentration of defects. This allows its physicochemical properties to be adjusted by doping and the insertion of several transition metals with various oxidation states at the spinel structure sites. The doping of Co3O4 with nickel improves the electrical conductivity, which is related to the occupation of the doping site and its state of valence [16]. Fig. 2 shows the substitution of nickel at sites 2+ and 3+ of Co3O4. When nickel is added, it substitutes preferably the octahedral sites of Co3+ through stabilization at sites 2+ and 3+ [17]. According to Koneru et al. [18], Ni2+ at the tetrahedral sites leads to a normal spinel, while Ni2+ at the octahedral sites leads to an inverse spinel or a partial inverse spinel structure.
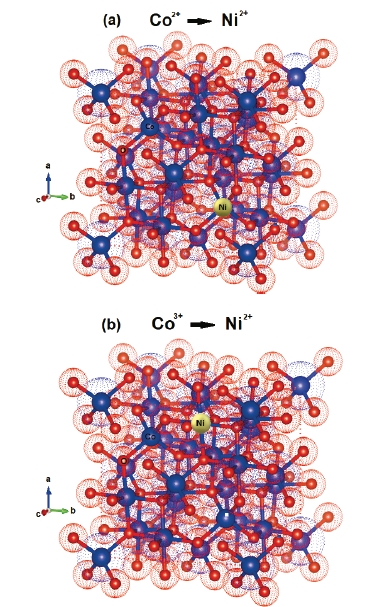
Source: The Authors using software Vesta.
Figure 2 Bulk structure of spinel Co3O4 with single tetragonal Co2+ cations (a) and single octahedral Co3+ (b), being replaced by Ni2+ cations.
Fig. 3 shows the X-ray diffraction (XRD) pattern of Co3O4 powder with the subsequent final heat treatment at 300°C for 3 hours and Co3O4 doped with Ni and calcined during the same time. In section (a), the precursors obtained by the hydrothermal technique and examined by XRD are crystalline, though it is difficult to assign the definitive pattern to a specific phase. This condition has been present in other deposits [13]. In section (b), Co3O4 was calcined at 300°C for 3h in air and the crystallinity of Co3O4 is observed. In section (c), the pattern corresponds to the synthesis of Co3-xNixO4 x=0.04, where the precursor peaks are observed again. Finally, in section (d), the pattern corresponds to Co3-x Nix O4 x=0.04 after final heat treatment at 300°C-3h, where the intermediate peaks disappear completely and other diffraction peaks correspond to crystalline non-detected products. This indicates the high purity of the final heat treatment product.
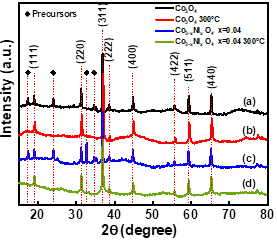
Source: The Authors.
Figure 3 X-ray spectra (XRD) for the Co3O4 samples obtained via hydrothermal technique: (a) Co3O4, (b) Co3O4 300°C (c) Co3-x NixO4 x=0.04 and (d) Co3-x NixO4 x=0.04-300°C.
All the powders obtained show diffraction peaks coinciding with the Co3O4 cubic spinel structure (space group F-4 3m) with file COD 00-001-1152. This agrees with other researches [13,19,20]. The XRD pattern with reflection peaks is associated with the planes (220), (311), (222), (400), (422), (511) and (440) at 2θ = 31.27°, 36.88°, 38.62°, 44.81°, 55.75°, 59.45° and 65.38°, respectively, and with the plane〈311〉as preferential orientation. The 2θ position peaks observed in (a) and (c) at 24.01°, 32.69°, 34.69° are associated with the precursors used in the synthesis as (Co(C2H3O2)2∙4H2O cobalt acetate. According to Singhal et al. [21], when the doping of nickel is carried out in Co3O4, a slight displacement is observed in the diffraction peaks.
Table 1 shows the energy-dispersive spectrometry (EDS) analysis with the percentages of atomic concentration. Results indicate the presence of elements of Co3O4 calcined at 300°C, as well as Co3O4 doped with nickel and calcined. Elements such as Co, O, C and Ni appear according to the synthesis of each of the compounds.
In order to establish structural parameters and properties, Rietveld refinement was carried out with the experimental data using the GSASII code. Fig.4 (a) corresponds to Co3O4 and Fig. 4 (b) to Co3-xNixO4 x=0.04- 300°C. The quality of the refinement can be checked through the values of the main refinement parameters: x2=1.25 and 1.26, Rwp= 0.89%, and 1.17% for Co3-xNixO4 x=0.04-300°C. There is a good correspondence between the simulated and experimental pattern. Table 2 shows the lattice parameters of the refinement and in Table 3 the positions of Co3O4 are observed. The refinement allowed to determine that Co3O4 crystallizes in a spinel structure with a spatial group F-43m and with lattice parameters a=b=c=8.092630Å. The refinement parameters are shown in Table 4, where values of GOF 0.90 and 1.13 are observed for Co3O4 and Co3-xNixO4 x=0.04-300°C, respectively. It has been observed that the lattice parameter and the unit cell volume for Co3O4 are close to those found in the literature [22-24]. According to Mulinari et al. [25] the variation in the observed atomic positions in the oxygen atoms may be associated with the formation of distortions in the bonds [O-Co-O].
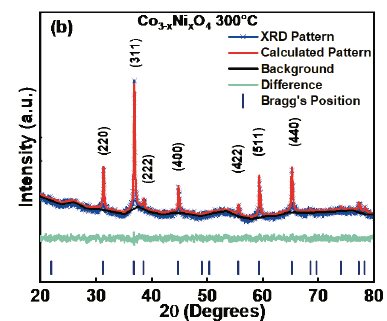
Source: The Authors.
Figure. 4 XRD refined pattern for the Co3O4 -300°C. Blue symbols represent the experimental diffraction data. The continuous line is the calculated pattern and the difference between the experimental and calculated patterns is represented by the base line.
3.2. Surface microstructure and composition
In Fig. 5, (a) and (c) indicate the SEM images of the precursors and (b) and (d) the products of Co3O4 after final heat treatment. In (a), there are particles with semicircular forms, most of them well defined. In (b), agglomerations appear with certain rods in some of the particles. In (c), the rods are notorious due to their diameter, and there are several particles. In (d), the structure is rather rough and has no definite shape. Irregular shapes are appreciated with agglomerations and certain inclusions that are attributed to nickel. The different forms obtained at 300 ° in (b) and (d) at 300°C are associated with the addition of nickel in the dopage in (d). Depending on the concentration of reagents, Co3O4 nanoparticles with different shapes can be obtained [26].
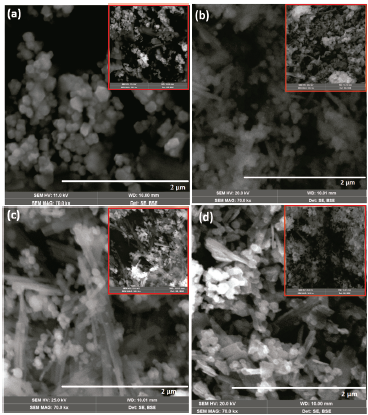
Source: The Authors.
Figure. 5 SEM Images (a) Co3O4 (b) Co3O4 -300°C(c) Co3-xNixO4 (d) Co3-xNixO4 -300°C.
It is assumed that temperature can affect surface morphology. According to Rajeshkhanna et al. [27], different sizes of anions embedded in the reaction mixture are responsible for the formation of different morphologies during the growth of the cobalt oxide material. The preferential adsorption/desorption of anions in specific crystallographic planes controls the formation of different morphologies, as well as the decrease in their surface energy.
Fig. 6 shows the process for synthesizing nickel-doped cobalt oxide. Initially, the mixture of nickel and cobalt is constantly stirred, with subsequent heating in the autoclave. Then, it was washed and dried.
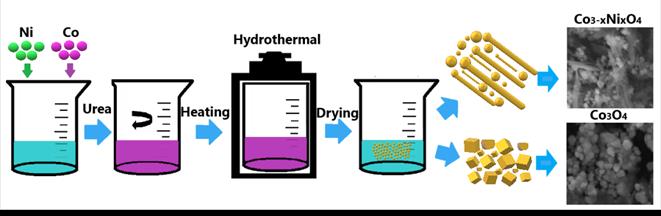
Source: The Authors.
Figure. 6 Schematic illustration of the fabrication process of Co3O4 - Co3-xNixO4.
As a result, nanostructures with semicubic and irregular rods were obtained. The autoclave was heated at 180°C for 20h (3°C/min), drying was carried out for 7h at 60 ° C in an oven, and the powders obtained were finally calcined in air at 300°C for 3h (10°C/min).
Organic and inorganic additives can be used to allow the precise control of various reaction parameters in the resulting composition, shape and size of nanocrystals, thanks to the liquid phase synthesis [28-30].
The average particle sizes were calculated by using the Debye Scherrer equation (0.9λ / (βcosθ)) and obtaining the average crystallite size. The term λ is the wavelength, from full width to maximum half (FWHM) of the diffraction peak, and θ is the Bragg Angle. The average particle size was calculated by using the most intense peak (<hkl>), (311). The crystallite size for Co3O4 and Co3-xNixO4 x=0.04 was found at an average of 41.575nm with a slight decrease when nickel is added. For Co3O4 at 300° C, the crystallite size decreases approximately 8 nm compared with Co3O4 without final heat treatment and with a value of 33.85nm, and Co3-xNixO4 x=0.04 300°C at 12nm with a value of 29.89nm, as shown in Table 5. These results agree with the works of Singhal et al. [21,31] where the peaks represent a good crystallinity and they obtained a crystallite size of 36-42nm.
These results can also be associated with the research by Lakehal et al. [10], who prepared films and fine powders of nickel-doped cobalt oxide on glass substrates. They used an immersion coating process based on sol gel with a concentration of Ni in the range from 0 to 9% by weight (%). The crystallite size of the samples was in the range of 214-279 Ǻ. According to Itteboina et al. [32], the heat treatment has an important role in particle size. Additionally, the crystallite size may be directly related to the nucleation table [33].
Fig. 7 shows the particle diameter size for (a) Co3O4 that corresponds to 20.73nm and (b) Co3-x NixO4 of 30.64nm. This size increases when cobalt oxide is doped with nickel. The difference is approximately 9.91 nm. It has been found that nickel substitution improves cobalt-spinel oxide activity by enlarging its specific surface area, its conductivity and its roughness factor, which is also called electronic and geometric effect [34].
3.3. Optical band gap determination
Fig. 8 (a) shows the UV-Vis diffuse reflectance spectra of Co3O4 alone and doped with 4% nickel, both calcined at 300°C. In the spectrum, the bandwidth values were calculated for the four samples shown in Table 6. The Eg1 value found is Eg1 = 1.51eV and correspond to O-2 → Co3+ [35], while for Eg2, the value is Eg2 = 2.74eV. For the Co3O4 doped with 4% Ni, there is a slight increase in the band gap compared with pure Co3O4. The gap energy changed from 1.51eV for pure Co3O4 to 1.55eV for the Co3O4 doped with 4% Ni. For the calcined Co3O4 doped with 4% Ni, there was also an increase compared with Co3O4 at 300 ° C (1.53eV for Co3O4 at 300°C and 1.56eV for Co3O4 at 300 ° C with 4% Ni). The highest bandwidth energy changed from 2.74eV for Co3O4 to 1.54eV for Co3O4 at 300°C. This behavior can be attributed to the lattice distortions caused by the temperature change, as well as to the introduction of nickel ions in the Co3O4 matrix and the formation of impurity energy levels (acceptor level) [10]. The morphology and structures of the materials are closely related to their optical properties, which are more evident in nanomaterials [36].
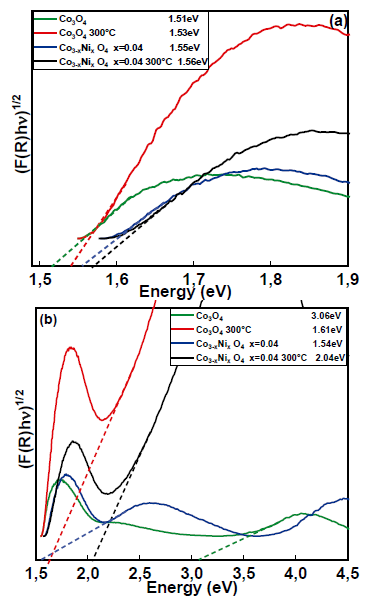
Source: The Authors.
Figure 8 Determination of the band interval for Co3O4, Co3O4 at 300°C, Co3-x NixO4 x=0.04 and Co3-x NixO4 x=0.04 at 300°C for (a) the first region and (b) the second region.
4. Conclusions
Compounds of Co3O4 and Co3-x NixO4 with nickel doping were synthesized and the subsequent final heat treatment was performed at 300°C through the hydrothermal technique. After heat treatment, the results obtained indicated the crystallinity of Co3O4 without the presence of Ni or NiOx impurities, according to the X-ray diffraction analysis. In the Co3-x NixO4 compound, the heat treatment at 300°C allowed the conversion of the precursor to particles.
Structural characterization in samples of Co3O4 and Co3-x NixO4 was found through Rietveld refinement of experimental X-ray diffraction data. This evidenced that the samples initiate its major crystallization into a cubic structure belonging to the spatial group F-43m (# 216) with cell parameters a=b=c= 8.092630 Å with a slight variation in the volume value of the unit cell and a lower value for the nickel-doped compound of 529.584Å. The particle size varies between 20 and 30nm. Optical band gap measurements allowed to establish the value of Eg1 and Eg2 between 1.51 and 2.74eV for the samples of Co3O4 calcinated and with doping.