INTRODUCTION
Insects are of extreme ecological relevance because of their enormous diversity and their important role as providers of ecosystem services and in ecosystem functioning (Losey and Vaughan, 2006; Weisser and Siemann, 2008; Cardinale et al., 2012). Insects have as well economic and medical significance as a wide range of agricultural pests, invasive organisms and disease vectors are insects species (Smith, 1973; Pimentel et al., 2005; Costanza et al., 2011). For instance, it was estimated that the annual control of a single insect pest species can cost as much as US$ 5 billion (Zalucki et al., 2012).
Nowadays, insects are a matter of debate in several topics of broad scientific and public interest. On the one hand, the decline of naturally occurring insect populations has raised awareness about the urge to preserve natural habitats and reduce the factors that cause these negative effects (Potts et al., 2010; Hallmann et al., 2017; Sánchez-Bayo and Wyckhuys, 2019). On the other hand, ongoing global spread of pest and invasive insect species impose a challenge for agricultural production (Bebber et al., 2014; Luque et al., 2014). In particular, crop losses to insect pests can be as high as 80% of the production (Oerke, 2006). In addition to the topics mentioned above, insect are also a current topic in the food-production industry as a vast amount of studies propose mass-reared insects as a viable alternative source of proteins for animal and human consumption (Van Huis, 2013). Studies in this matter range from rearing techniques, up to nutrient content and perception of the product by the consumer (Megido et al., 2016; Barsics et al., 2017).
All the aforementioned arguments on the current importance of insects support the need to deepen the understanding of responses that these outstanding organisms (i.e. insects) may elicit to stressors and ongoing environmental changes. An interesting example of the impact that insect responses can have on the human-environment interaction is the termed “pollinator crisis” (i.e. decline of honey bee and bumble bee populations). This phenomenon is occurring at a global scale and has motivated changes in the legislation of several countries in an attempt to control or reduce the major stressors that may be causing this critical situation (e.g. parasites, pesticides and loss of flowering plant diversity) (Potts et al., 2010; Hall and Steiner, 2019). However, that is not the case for most of the contemporary environmental problems related to insects. The effects of well-known environmental stressors (so called drivers of global change), such as increased CO2 and global warming, have been extensively studied in controlled (e.g. lab) and field conditions (Bidart-Bouzat and Imeh-Nathaniel, 2008; Scherber et al., 2013). However, many other stressors are relatively less studied. For instance, synthetic chemicals (e.g. pesticides) have been known to affect non-target insect species since long ago (Ware, 1980; Pimentel, 1995). Yet, only recently have been considered as significant drivers of global change (De Laender et al., 2016; Bernhardt et al. 2017; Sánchez-Bayo and Wyckhuys, 2019) and their interactions with ecosystem processes are starting to be unravelled (Schäfer et al., 2007).
The studies on the responses of insects to environmental stressors are still scarce and the majority of them have focused on numerical declines without analysing the underlying mechanisms (Kaunisto et al., 2016). Besides, most studies carried out at large scale considering community dynamics in responses to changes in the environment often overlook the responses at the individual level (Todgham and Stillman, 2013; Schulte, 2014). The mechanistic basics of responses to stressors are still poorly understood (Kaunisto et al., 2016) and this is further aggravated by emerging environmental stressors in managed and natural ecosystems (e.g. pollution and biological invasions) caused mainly by human activities (Todgham and Stillman, 2013). Although some authors have started to point out the physiologically-related causes of the sensitivity of insects to stressors (Klein et al., 2017), this is still a topic highly disregarded for the vast majority of extant insect species (Kaunisto et al., 2016; Urban et al., 2016).
Environmental Stressors
Environmental stressors can be defined as factors that hinder the achievement of optimal condition, either by acting as constraints (in relation to biological demand) or by causing detrimental effects on organisms because of increased or extended exposure (Schulte, 2014; Freedman, 2015). A range of biotic and abiotic factors can be included in such definition: from climatic stressors (e.g. temperature and humidity (Sinclair et al., 2003; Musolin, 2007)), up to biological (e.g. trophic interactions and competition (McCauley et al., 2011; Adamo et al., 2013; Gutiérrez et al., 2020a)) and chemical stressors (e.g. heavy metals and pesticides (Tchounwou et al., 2012; Bohnenblust et al., 2013; Gutiérrez et al., 2016; Gutiérrez et al., 2017a; Gutiérrez et al., 2017b; Gutiérrez et al., 2020b; Bernhardt et al., 2017)). Moreover, these evidently bear the potential to affect all living organisms, from bacteria to vertebrates, and their associated ecosystem functions and processes.
It has been recognized that in natural conditions, organisms rarely experience optimal environments because biotic and abiotic factors fluctuate at a moderate extent (Steinberg, 2012; Wingfield, 2013). During their lifetime, organisms are often exposed to a variety of environmental stressors that would not be experienced individually, but rather in a mixture that interact in complex and dyamic ways (Schulte, 2014; Côté et al., 2016).
The need for experimentally addressing the effects of the interacting stressors stressor was recognized several decades ago (Breitburg et al. 1998) and nowadays is considered a topic of substantial importance for ecosystem management and conservation (Todgham and Stillman, 2013; Craig et al., 2017).
¿How do organisms respond to environmental stressors?
Environmental stressors can, depending on their magnitude and intensity, trigger a variety of response reactions in living organisms (Figure 1) (Pimentel, 1994; Koolhaas et al., 2011). In essence, organisms would try to maintain stability through change (Romero 2004), that is to say; they to say, they would try to (at least partially) compensate for fitness reduction caused by a harsh environment (Schulte, 2014). And such responses can span through several levels of biological organization, from behaviour to physiology, morphology and ultimately the genome (Kassahn et al. 2009; Gutiérrez et al., 2020a).
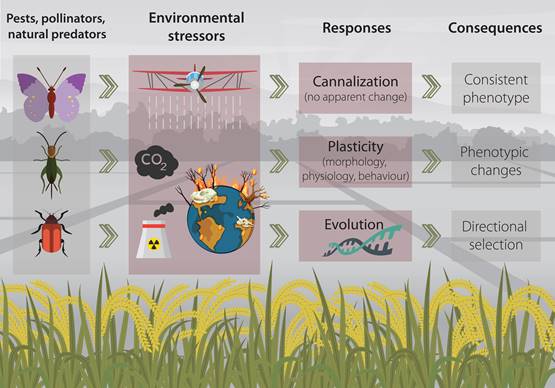
Figure 1. Conceptual figure of the mechanisms that agricultural insects may employ when facing common environmental stressors in agroecosystems.
Within the organisms’ machinery for facing environmental stressors, some traits may remain unchanged while others can be highly plastic. Usually, physiological and behavioral traits (considered at the individual level) are more prone to quick response as they can be modified in a short time-scale, conversely to morphological traits that would require a considerable longer time to respond to the environmental cues (Padilla and Adolph, 1996; Gutiérrez et al., 2020b).
Some organisms may employ a mechanism termed canalization, through which they achieve a consistent phenotype regardless of the stress imposed by the environment (Waddington, 1942; Debat and David, 2001). While traits important to fitness are considered to be strongly canalized (Stearns and Kawecki, 1994), in some cases, this “response” (or the lack of it), can be simply the “insensitivity of a character to environmental factors” (Wagner et al., 1997). However, canalization is only considered advantageous in homogeneous and stable environments as the development of consistent phenotypes regardless of environmental changes (e.g. stressors) may be maladaptive (Zabinsky et al., 2018).
A second mechanisms for coping with stressors occurring within an organism’s lifetime is phenotypic plasticity (Gabriel, 2005; Schulte, 2014). Which implies the modification behavioral, behavioural, physiological, morphological and developmental traits to successfully overcome changes in the environment (Callahan et al., 1997). Classical examples of plastic responses are the morphological and behavioural changes in Daphnia (Cladocera, Daphniidae) induced by predator-induced stress (Boersma et al., 1998), and the capability of Schistocerca gregaria (Orthoptera, Acrididae) to generate three types of polyphenism (which implies morphological and physiological changes) depending on environmental and visual cues (Tanaka et al., 2016).
It is important to consider that the distinction between canalization and plasticity can be challenging because some traits may remain stable as they are buffered by a different mechanism in an attempt to maintain homeostasis (therefore, plasticity could only be traced by monitoring multiple components of the phenotype). Moreover, living organism may simply opt for remaining unchanged despite the challenges imposed by the environment (Wagner et al., 1997). On the other hand, some organisms are “compelled” to remain unchanged simply because they may have a very limited plastic capacity (DeWitt et al., 1998), such “limitation” is thought to be (at least partially) explained by genotype (Metcalfe and Monaghan, 2001).
Finally, as a third mechanism, organisms can respond to the stress imposed by the environment through evolution. In this scenario, the environment would drive the selection pressure for the development of population divergence and even speciation (Bijlsma and Loeschcke, 2005; Lexer and Fay, 2005). A common example of this type of directional selection (in the context of environmental stressors) is the evolved resistance to antibiotics, heavy metals and pesticides (Davies and Davies, 2010; Gutiérrez et al., 2019; Gutiérrez et al., 2019).
The evolution of mechanisms to overcome environmental stressors (e.g. pesticides) may provide the means to survive and reproduce in hostile environments (e.g. highly managed agroecosystems) (French-Constant et al., 1999; Gutiérrez et al., 2020b). However, such mutations commonly have associated negative consequences for the fitness of the species due to physiological trade-offs (Coustau and Chevillon, 2000; Haubruge and Arnaud, 2001).
Outlook and recommendations
Although some studies have attempted to model responses to stressors based on other known effects or biological traits (Baird and Van den Brink, 2007; Haddad et al., 2008; Diamond et al., 2011). Experimental data, especially for interacting factors, is still much needed as it has been pointed out that biological responses are difficult to predict (Davis et al., 1998; Angert et al., 2011). For instance, some empirical studies have demonstrated the complex interactions that environmental stressors can exhibit, from additive and synergistic, to antagonistic and even reversed (Jackson et al., 2016).
While studying the effects of multiple stressor is challenging due to operational constraints (resources, space and workload), it remains as the most reliable way to elucidate the complex interaction of the studied factors (Todgham and Stillman, 2013; Schulte, 2014; Côté et al., 2016). Furthermore, it is fundamental to study the responses to stressors in long-term experiments (relative to the life cycle of the model organism) as the sensitivity or responsiveness of the organisms may vary according to the life stage (Metcalfe and Monaghan, 2001). It would be interesting to undertake further research in two contrasting, yet complementary, directions: 1) more controlled and mechanistic approaches to understand and unravel effects of environmental stressors at the individual level. 2) A larger scaled multi-species approach to study effects that can scale up to the community and ecosystem level (mainly related to structural and functional attributes).
CONCLUSION
Understanding insect responses and the mechanisms involved (e.g. plasticity and evolution) would provide the necessary tools to dissect environmental (e.g. biodiversity loss), economic (e.g. pests and insecticide resistance) and public health (e.g. insect-borne diseases) problems given that stressors may ultimately affect ecosystem functions and processes (Christensen et al., 2006). Additionally, as mentioned above, plasticity exhibited by living organisms can significantly affect ecological patterns and processes (Miner et al., 2005). Therefore, it would be expected that community dynamics, food webs and ecosystem processes are responsive to change in, for instance, behavior and resource investment of particular species. Besides, studying the response as a whole of synthetic and natural communities to interacting environmental stressors is a promising line of research (Steinberg, 2012; Craig et al., 2017).