Introduction
Colombia is the only Latin American country where the number of antipersonnel landmines (APLs) buried has increased in recent years [1]. This increase is due to the use, by illegal armed groups, of APLs as part of a strategy aimed to prevent access of law enforcement authorities to areas where illegal economic activities occur (e.g., coca plantations, drug storage, clandestine cocaine processing laboratories, guerrilla camps, among others) [2].
Considering the recently signed peace agreement between the Colombian government and Colombia's largest and oldest insurgent group (FARC-EP), as well as on-going peace negotiations with other organized illegal groups, it is expected that the manufacture of APLs will eventually decrease.
The lack of precise information about the location and number of buried APLs, their increased use by drug traffickers, and technical weaknesses in currently-used detection procedures evidence an urgent need to engage in research efforts. In this way, it is necessary to generate new detection strategies, new sensors, and to improve demining practices that could eventually lead to a territory free of APLs [3]. As a clarification, due to their artisanal manufacturing, these explosive elements can be named as improvised explosive devices (IED), making their detection difficult. According to their course of action, they can also be classified as APL since any living being close to the device can activate them and become a victim [4,5].
According to Centro Nacional Contra Artefactos Explosivos y Minas - CENAM (National Center for Explosive and Landmines ) [6], about 99% of the APLs found in Colombia are based on ANFO (96% ammonium nitrate and 4% fuel oil). This mixture is made since the explosive properties of ammonium nitrate are improved with the addition of fossil fuel. When hydrocarbons are mixed with ammonium nitrate, the decomposition reaction of ammonium nitrate becomes more exothermic, from ΔH= -36 kJ mol-1 to ΔH= -280 kJ mol-1 [7].
Illegal groups take advantage of the agrochemical accessibility to make ANFO in an artisanal way. This since they know the ANFO explosive power and that most of the fertilizers used in Colombia are based on ammonium nitrate. The inorganic salt, ammonium nitrate, is the major component of ANFO. This salt presents a high hygroscopicity that, under atmospheric conditions, produces a reaction that releases vapors of ammonia and nitric acid into the atmosphere [8]. For the purposes of ANFO detection means that these vapors can be used as target analytes. The majority of detection methodologies for APLs have been focused on the detection of ammonia [10] because a higher concentration of ammonia is expected near ANFO-based explosive devices [9]. This due to the vapor pressure for ammonia and nitric acid, 8.6 x 105 and 3.3 x 103 Pa, respectively.
The objective of this review is to describe strategies for the detection of ANFO-based APLs. We also report on technological advances pioneered in Colombia as the country engages in a massive demining endeavor. The document is composed of three sections; the first one addresses different techniques used in the detection of explosives. A second section describes worldwide and Colombian efforts related to APLs detection. The last section presents the use of trained canines for the detection of APL and how some environmental and manufacturing factors of the explosive affect the detection by them.
Analysis of explosive compounds
The detection of explosives, unexploded ordnance, and the establishment of security protocols for public places and demining techniques are active research topics for academic, private industry, and governmental entities. This has led to the publication of a large number of reviews in various areas, among which are the properties to consider in explosives for the development of detection and analysis techniques [11,12], the detection of traces and vapor of explosives [13], explosive screening and extraction [13], and the development of explosive analysis methodologies by ion spectrometry (including mass spectrometry and ion mobility spectrometry) [14], ion chromatography [15], and capillary electrophoresis [16]. In general, the detection of explosives has two goals: a) preventive, in which the purpose is to find the explosive material before its detonation, and b) forensic, which seeks to study post-explosion traces to identify potential suspects to a crime scene [17].
Study and identification of explosives by analytical methods
Forensic chemistry has shown that analytical techniques are very efficient tools to identify explosive compounds and of fundamental importance in the development of highly sensitive sensors [18]. Among these techniques, we highlight gas chromatography (GC), high-performance liquid chromatography (HPLC), ionic mobility spectrometry (IMS) and mass spectroscopy (MS).
Despite the progress in explosive detection, few studies have addressed in explosives based mixtures, as ANFO, compared with organic explosives. In the case of ANFO, analytical techniques have been focused on the detection of ammonium nitrate or some fuel oil. For example, Benson et al. [19] demonstrated that isotopic relations mass spectroscopy (IRMS) was capable of differentiating three ammonium nitrate samples manufactured by three different Australian companies. This identification was achieved by analyzing stable Oxygen and Hydrogen isotopes.
Flanigan et al. [20] used laser electrospray mass spectrometry (LEMS) and principal component analysis (PCA) on three samples: Black powder (before and after an explosion), ammonium nitrate, and a mixture composed of chlorate, perchlorate, and sugar. Through PCA, the researchers were able to group the results into three distinct categories that were representative of the chemical composition of each explosive compound (Figure 1).
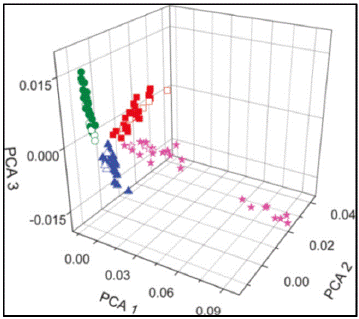
Figure 1 Three-dimensional projected PCA analysis for the chlorate-perchlorate-sugar mixture (red), ammonium nitrate (green), black powder before the explosion (magenta), and black powder after the explosion (blue). Reproduced with permission from ref [20]. Copyright 2011, American Chemical Society.
Taking into account explosive attacks on automatic teller machines (ATMs), Hernandes et al. [21] studied the presence of ANFO on banknotes. To designate the diagnostic ions, high-resolution mass spectrometry with two analyzers, ion cyclotron resonance (ICR) and quadrupole ion trap (QIT), was used as the analytical technique. With the ESI (-) mode, three ANFO diagnostic signals were found before and after the explosion, where the most important and decisive one presented a m/z of 210 corresponding to the cluster [(NO3)2 24Mg]-. Additionally, the authors studied a novel technique that allowed the analysis of ANFO on bill surfaces. This technique uses an ionization mode, and a sonic spray generated at ambient conditions (easy ambient sonic-spray ionization, EASI). Then, confirming the presence of nitrate ions (m/z = 212) and the marker cluster [(NO3)2 24Mg]-, which allows reliable detection of ANFO residues on any surface.
During the last decade, the direct analysis in real-time (DART) has demonstrated the ability to generate chemical ions from compounds present in crime scenes [22]. Forbes et al. coupled infrared thermal desorption (IRTD) and real-time mass spectrometry (DART-MS) to detect organic and inorganic explosives, including ammonium nitrate [23]. This technique makes possible the thermal desorption of volatile and semi-volatile organic explosives at relatively low temperatures. Similarly, non-volatile inorganic explosives such as potassium chlorate and potassium perchlorate were analyzed but using high temperatures for the desorption. The results showed sensitivities in the order of nanograms for several inorganic oxidants and of sub-nanograms for common organic explosives based on nitrates.
Another spectroscopic technique widely used for the identification of explosives is Raman spectroscopy [24]. Yellampalle et al. identified 15 explosives thanks to the variations observed in the intensities of the characteristic Raman bands according to the excitation wavelength used [25]. Specifically, for ANFO, the Raman spectra show significant variations in the bands located at 1042 and 1378 cm-1. In the same way, Almeida et al. applied Raman hyperspectral methodology, with independent component analysis (ICA), to detect real ANFO samples after an ATM explosion. This approach allowed the identification of ANFO in small quantities (70 p_g cm-2) present in bills and other related samples [26].
Other strategies explored in the detection of explosives are nuclear quadrupole resonance (NQR) and differential thermal analysis. In NQR, the existence of an electric quadrupole moment [27] was used by Rudakov to develop a multi-pulse technique useful in detecting ANFO and RIOGEL (industrial explosive with 60% nitrate of pure ammonium) type explosives [28]. In turn, Nazarian et al. used differential thermal analysis [29] and reported the thermochemical characterization of two explosive mixtures: ammonium nitrate - nitromethane and ANFO. The changes observed in the thermograms of ANFO samples with different preparation times were attributed to the variable volatility of the hydrocarbons in fuel [30].
Considering that the majority of explosives contain C-H or N-H bonds in their structure, Zapata et al. delved into using near-infrared spectroscopy (NIR) on 18 different explosives. They found that for explosives based on ammonium nitrate, some bands are observed in the 1900 to 2200 nm and from 1450 to 1650 nm regions [31]. For ANFO, bands corresponding to the C-H bonds of the fossil fuel were not detected due to their low proportion.
Recently, neutron activation analysis has emerged as a strategy to determine the elemental composition of explosives. This, thanks to the gamma rays emission that characterize the interactions between neutrons and nuclei of atoms present in explosive compounds [32]. Taking this principle into account, Cevallos et al. simulated three configurations varying the geometry of the device, material thickness, number, position, and size of the gamma-ray detectors. Using Monte Carlo N-Particle methods (MCNP6), the simulations studied two different scenarios: blank and ammonium nitrate. The results demonstrated that the best configuration corresponded to the smaller cavity, which increases the neutron flux [33].
Detection of Explosives by nanostructured devices
Considering the vapor pressure of nitro explosives, including ANFO, the commercially available technology known as TrueTrace®, designed by FLIR Systems, offers portable devices (Fido® X2 and Fido® X3) based on fluorescent polymers that quenched the fluorescence after interacting with the vapors released by the explosive (Figure 2). Besides, FLIR Systems offer the Fido® X80. This is a product based on mass spectrometry that analyzes personal objects, packages, skin, and vehicles. The Fido® X80 is an ideal sensor for detecting explosives in airports, considering the research work by Swager [35] as a founder of FLIR systems. It is expected that Fido® X2 and Fido® X3 are designed to detect nitro explosives, including ANFO.
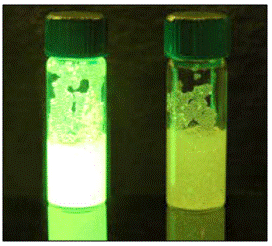
Figure 2 Quenching (right) caused by the presence of vapors released by explosives. Taken from TrueTrace® website [34].
Most recently, and following the work by the Swager group, fluorescent co-polymers [36] and metal-organic frameworks (MOFs) have been studied as fluorescent sensors [37-39] in the detection of nitro explosives. Sheykhi et al. reported the detection of explosives based on nitromethane and ammonium nitrate, using fluorescent polymers. They found a quenching effect on the sensor in the presence of nitromethane, and an increase of emission intensity in the presence of ammonium nitrate, a component of ANFO explosive. The fluorescence behavior was attributed to the nitroalkene complex formation and the protonation of free amines together with the hydrolysis of the imine bond [40].
Considering the sensitivity and selectivity of MOFs as sensors, Travlou et al. demonstrated that MOFs are good candidates as chemosensors for ammonia. In their work, a hybrid material composed of MOF (copper/ benzene tricarboxylic acid) and graphene oxide, when exposed to different concentrations of ammonia, showed linear changes in the electrical resistance (Figure 3). However, this material presented moisture-induced instability [41].
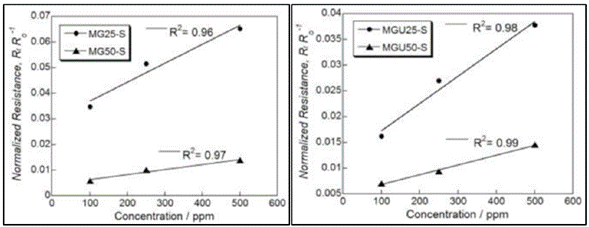
Figure 3 Change dependence on the normalized resistance of MOF hybrid materials in relation to the ammonia concentration. (M = MOF, G = graphene oxide, GU = aminated graphene oxide, 25/50 = % graphene in the hybrid material). Reproduced with permission from ref. [41]. Copyright 2015, Royal Society of Chemistry.
Campbell et al. reported on a MOF of copper and 2,3,6,7,10,11-hexaimino triphenylene (HITP), which detected ammonia concentrations in the order of 0.5 mg L-1 at a relative humidity of 9% (Figure 4) thanks to changes in its electrical conductivity. Unfortunately, when the humidity increased to 60%, simulating real conditions, it was only possible to reliably detect ammonia concentrations of 5 mg L-1 [42].
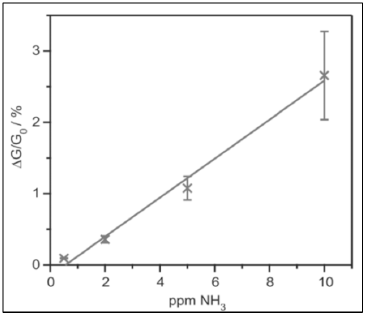
Figure 4 Electrical response of the MOF-Cu chemosensor to different concentrations of ammonia. Reproduced with permission from ref. [42]. Copyright 2015, John Wiley and Sons.
Currently, a team of researchers from the University of Cranfield, Universidad Nacional de Colombia, and the University of Oxford are collaborating to develop optoelectronic sensors. This by synthesizing MOFs comprising acetates or chlorides, cobalt, and copper, with derivatives of terephthalic acid. These MOFs have shown colorimetric changes in response to aqueous solutions of 100 mg L-1 ammonia. The team is working on increasing the sensitivity and selectivity of these materials using new organic ligands [43].
In 2017, MOF structures were reported as post-explosion photoluminescent markers for the detection of ANFO-type explosive residues. In this study, three materials were compared: two MOFs and one ceramic. Both MOFs had pyridine-2,6-dicarboxylic acid (DPA) as ligand and mixtures of lanthanum / terbium (material 1) and lanthanum / terbium / europium (material 2) as metal centers. The ceramic material consisted of zinc oxide of composition ZnAl195Tb05O4 (material 3). To each marker, 10 g of ANFO was added in three different weight ratios Mmarker/MANFO: 1, 3, and 5%. The emission spectra and the images of post-explosion residues collected in materials 1 and 2 (Figure 5) showed characteristic peaks when used proportions greater than 3.0%, allowing the unambiguous identification of the ANFO explosive. For material 3, no luminescent residues were observed, which demonstrates that MOFs could be used in the efficient identification of ANFO explosives [17].
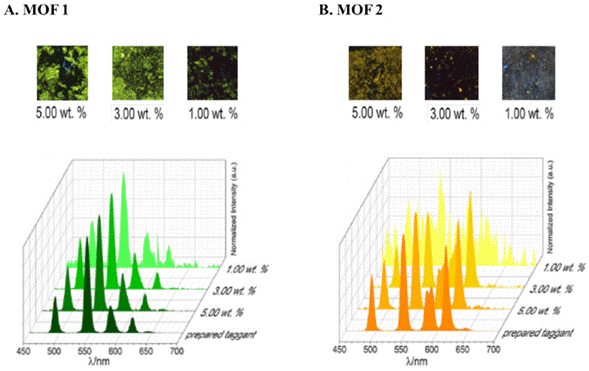
Figure 5 Video spectral comparator (VSC) emission spectra of prepared markers and post-exploded debris collected from MOF 1 (A) and MOF 2 (B). Differences between MOF 1 and MOF 2 are the La3+, Tb3+, and Eu3+: 1.6/0.4/0 mmol and 1.6/0.2/0.2 mmol, respectively. Adapted from Mauricio et al. [17].
A sensor based on the concept of 'electronic nose' that uses metal oxides to detect ammonia in ANFO explosives was developed by Silva et al. [10]. The researchers demonstrated that the right candidate for the detection of ammonia is zinc oxide. However, CO2 filtration is required since it presents the same response pattern as the analyte of interest.
Analysis of volatile organic compounds (VOCs) released by ANFO explosives
Before carrying out the identification of VOCs released into the atmosphere by an explosive, the collection and concentration of these compounds must be carried out. In this way, the analysis of the sample will be the most adequate and reliable, and the results will be consistent with the actual composition of the analyzed matrix. There are different extraction techniques for the collection of analytes. For ANFO, the most used has been the passive Ogawa samplers, which use pads covered by a sorbent to collect ammonia [44]. Another pretreatment technique reported for ANFO sampling is solid-phase microextraction (SPME), which is adequate for low detection limits, low cost, easy handling, and solvent-free extraction [45]. Brown et al. applied headspace (HS) SPME to analyze Riogel 2 and Nitrex, ammonium nitrate-based explosives on gas chromatography coupled to mass spectrometry (GC-MS). In this study, two compounds in the explosives were identified: ammonia (from ammonium nitrate) and methylamine (from methylammonium nitrate). These results reveal the importance of identifying other substances besides ammonia in explosives like ANFO. This would allow differentiating between the ammonia released by a dangerous device or the originated by biological processes [46]. Lubrano et al. [47] resumed the advances found by Brown's team and quantified the ammonia in ANFO and ammonal (a mixture of ammonium nitrate and aluminum powder) as butyl carbamate through GC-MS, using diethylamine (DEA) as an internal standard, determining that the average concentrations of ANFO and ammonal in the HS were 6 ±2 µg L-1 and 21 ± 3 µg L-1 (Figure 6). In the two studies mentioned above, it is clear that the methodology shows good results under laboratory conditions. However, it is necessary to consider environmental conditions for the identification of ammonia in the field.
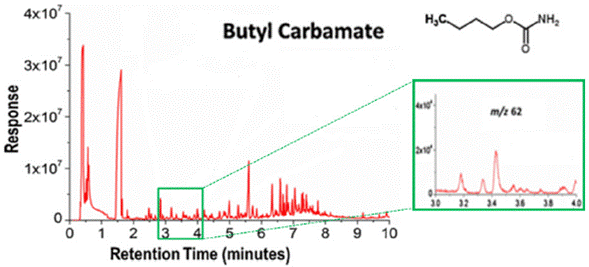
Figure 6 ANFO total ionic current. The zoom corresponds to the retention time of butyl carbamate, with the characteristic peak of m/z 62. Adapted from Lubrano et al. [46]
DeGreeff et al. published the analysis of different explosives, including ANFO, using HS-SPME/GC-MS as extraction technique, but making changes in the SPME fiber-coating polymer. Instead of using the fiber from the previous reports (polyacrylate-PA), they used a fiber with a triple coating of polydimethylsiloxane / divinylbenzene / carboxen (PDMS / DVB / CAR) [48]. With this modification, the spectra indicate that the majority of the ANFO specimen's peaks are due to hydrocarbon compounds derived from the fossil fuel in the mixture.
A new research compares volatile organic compounds released at different ANFO origins in simulated APLs by chromatography and morphological analysis using scanning electron microscopy (SEM) analysis. The results obtained in this investigation showed the first detection of primary amines (dimethylamine), other than ammonia, in homemade ANFO samples (Figure 7). Regardless of the origin of the material, these compounds are established as characteristic for ANFO. Additionally, morphological differences by SEM were observed according to the amount of fuel oil in each sample. These results suggested that through the combination of different techniques, it is possible to obtain fundamental information to understand the olfactory mark of ANFO with different origins. Thus, approaching the development of more efficient APL sensors based on ANFO [49].
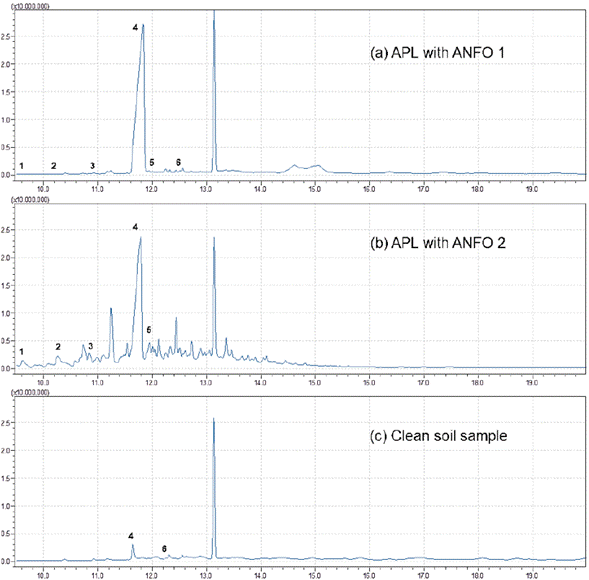
Figure 7 TIC obtained in the sampling of simulated APL with (a) ANFO 1 - material seized by the Colombian army, (b) ANFO 2 - a mixture of diesel and ammonium nitrate (extracted from a commercial fertilizer) manufactured in a laboratory, and (c) clean soil sample. Identified compounds: 1. Nonane. 2. Alkane. 3. 3-methylnonane. 4. Butyl carbamate. 5. 1,4-diethylbenzene. 6. Butyl dimethyl carbamate. Reproduced with permission from ref. [49]. Copyright 2020, John Wiley and Sons.
Recently, a study that combined GC-MS and FT-IR for ANFO samples, made with different kinds of diesel through a principal component analysis (PCA) and linear discriminant analysis (LDA), showed that the components farnesane, norpristane, phytane, and four fatty acid methyl esters (FAME) provided different degrees of discrimination of the ANFO samples. Additionally, this study demonstrated that chemometric analysis is a useful tool to find the differences among samples like ANFO explosives [50].
Analysis of explosive compounds
In addition to the wide range of chemical compounds that can be used in the manufacture of improvised explosive devices (IED), the complexity in the detection of APL increases by several factors. Among them are the manufacturer's creativity in new ingredients, the availability of materials in the area, the environmental conditions where they are placed and the type of activation mechanism. Figuli et al. compared the explosive power between industrial and home ANFO. The results showed that homemade explosives are 75% less effective than industrially manufactured ones. However, more than 95% of all terrorist attacks are carried out using homemade ANFO explosives [51].
The manufacture of APL and explosive devices is becoming more sophisticated and almost undetectable for methodologies commonly used in demining, such as metal detectors. Additionally, chemical activation systems are now being used, which unfortunately cause APLs to have longer lifetimes [52]. Some of the analytical techniques mentioned have also been used to detect APL. So that, they will be discussed again, along with other complementary strategies.
Mass et al. analyzed ANFO and pentolite (a mixture between TNT and PENT) both pure and mixed through Raman spectroscopy. The results of Raman spectra showed two characteristic peaks for the ANFO sample. They correspond to the bending in the plane (713 cm-1) and symmetrical stretching (1042 cm-1) of the nitro group, which is assumed as positive detection of ammonium nitrate. These results lead the authors to suggest the viability of a portable Raman as a tool for the detection and identification of explosive substances. However, there is a significant presence of noise in the signal each time the device moves from one place to another, requiring a constant spectrometer calibration [53].
In the search to improve the effectiveness of metal detectors, some techniques have been explored for APL detection. For example, ground penetration radar (GPR) consists in generating an image of the shape of an object found in the ground using radiation in the microwave region [54]. However, GPR systems have difficulties related to depth and speed delays in detecting APLs. As an alternative to solve these problems, Bayram et al. studied different algorithms to develop forward looking infrared (FLIR), images that allowed adequate detection of landmines taking into account the type of soil and the different shapes of the devices buried. Their results indicate that using FLIR images as prefilters for GPR systems increases the distance at which the sensing can be made and the speed of the data processing for the detection of landmines [55].
An example of humanitarian demining occurs in Donbass conflict zone (Ukraine). There, Bechtel et al. used a GPR for the electromagnetic characterization of soils and it was able to design suitable holographic impulse sensors. The authors indicated that the variation in electromagnetic properties was surprisingly small and within the normal range for most soils throughout the world [56]. About the development of holographic radar for the detection of landmines, Qin et al. modeled a monostatic antenna with a fixed frequency to transmit and receive electromagnetic signals, considering the conditions of flat and sinusoidal surfaces. The results showed that the theories of 'medium wavelength' and 'concave-convex lens' are useful in reducing image artifacts and obtaining the best quality data for the objectives detection and classification [57]. In Colombia, the manufacture of GPR-based sensors has also been explored as a complementary method to metal detectors. In 2005, a GPR camera was designed to obtain images and generate a probabilistic map of APL presence. Detection is based on the evaluation of soil texture and color variations, which reveal if the surface has been intentionally modified [58].
In 2014, a complementary tool was proposed for the detection of APL from the air [59]. Artificial vision from drones may be useful to geolocate the APL. This system was evaluated under three parameters: flight altitude, speed, and visibility of the object. Subsequently, they proceeded to evaluate the detection of APL for different types of terrain and visibility. The authors proposed to incorporate this technology into a robot to obtain a safer and smarter autonomous system to match the characteristics in demining missions in countries like Colombia [60].
In addition to the danger posed by APL detection, the variability in size and materials made their detection more difficult. A strategy to locate APL more effectively is the integration of electromagnetic induction (EMI) sensors. In this case, EMI is used for the location of small APLs containing metal, whilst GPR systems are used for the detection of large plastic APLs. Knox et al. explored linear regression and gradient increase fusion algorithms. This due to improving the results obtained in individual GPR or EMI systems when evaluating specific plate locations that simulated APL presence. It is expected that favorable results found will include soil information from the surrounding area [61]. Recently, this dual sensor was tested at the Universidad de los Andes in Colombia to evaluate the detection of APLs without metals, demonstrating progress in the processing of the signals emitted by the sensor [62].
The studies described above (excluding Raman detection) have focused on improving the capabilities of a metal detector. Nevertheless, these methodologies are inefficient when APLs do not contain metals. That is why other strategies aimed at detecting the chemical substances that the explosive form have been addressed. One of the investigations carried out in Colombia for the detection of ANFO-based APLs is based on nuclear quadrupole resonance (NQR). Cardona et al. focused on detecting ammonium nitrate by NQR, and it was able to detect this inorganic salt, both in laboratory and field conditions. Unfortunately, the signal is lost at depths bigger than 3 cm [63].
Another technique used in the detection of APLs is laser-induced breakdown spectroscopy (LIBS). This is a rapid chemical analysis technology that uses a short laser pulse to create a micro-plasma on the surface of the sample [64]. In 2009, it was reported that LIBS identified the ammonium nitrate present in soils (Figure 8) [64]. However, no ANFO experiments were done for safety reasons. Instead, tests were made with fuels without identifying their bands. Since fertilizers mostly contain ammonium nitrate, the detection of this inorganic salt in soils would cause interference in field test measurements aimed at detecting ANFO [65].
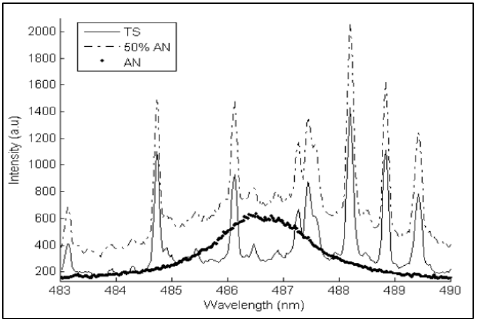
Figure 8 Spectra of ammonium nitrate (AN), soil (TS), and a mixture of 50% by weight of ammonium nitrate in soil. Reproduced with permission from Díaz et al. [65].
It is necessary to develop an efficient and reliable sensor for the detection of APLs and the use of different detection modalities that complement each other, such as the biological detection of explosives. In this field, the most used detector is canine. Still, other animal species (rats, pigs, and bees), plants (genetically modified), microorganisms, and enzymatic technology (nanodogs) have been proposed as alternative methods for this type of detection [66].
Taking into account the advances made by the African organization APOPO [67], in Colombia, the national police studied the effectiveness of laboratory rats in detecting APL. Still, the results of detection in real mined fields have not been published yet. The significant advantages of this methodology are the lightness of the rats since it is not enough to activate the APL and the species that can be reproduced anywhere in the world, mainly the Wistar [68].
Canine detection
Chemoreception is the process of detecting chemical compounds performed by living organisms. This process involves the interaction between olfactory neurons and VOCs, which are chemical compounds with moderate molecular weight, low polarity, high vapor pressure, lipophilicity, and particular water solubility. Currently, canines are the most widely used method of detecting explosives in real-time, thanks to their ability to locate odors from explosive material. Trained canines continue to be the model to compare upon which other explosive detection methods are evaluated. The canine olfactory system has exceptional sensitivity and selectivity, perceiving many different aromas at the same time, a feature difficult to reproduce artificially [69].
Interestingly, there is still scientific debate about the biochemical process that causes detection by the canine due to the need for psychophysical tests and the differences between dog species [70]. Two hypotheses have been raised: a) dogs detect the smell as vapor, and b) the smell is trapped and transported by particles that are inhaled [71]. Beltz explains that the olfactory activation process in the canine's nose begins when VOCs enter the olfactory system and bind to receptor proteins located in the phospholipid bilayer of the cell membrane [72].
Sargisson et al. assessed the environmental conditions that determine mines detection by canines trained in Afghanistan [73]. It was found that the landmine detection process is less efficient when the humidity is high. Additionally, APL efficiency detection is affected by the depth and vegetation of the surface where the mine is buried. Similarly, in a recent work on demining conducted by trained canines in Colombia, Prada et al. highlighted the environmental factors that may affect the speed and type of VOCs released by an explosive buried in the ground [2]. Among them, they identified the polymer type used as a mine container, the nature of volatile, and the environmental conditions in the area where the APL is located.
The book "Mine Detection Dogs: Training, Operations and Odor Detection", of the Geneva International Demining Center (GICHD), mentions that there is a high probability that the VOCs released by explosives are the product of the material in the explosive container (metal, plastic) and the degradation of the explosive on its way to the surface. This chapter also discusses the possibility that explosive molecules firmly adhere to soil particles suspended in the air or that these molecules get "activated" due to the canine movement when sniffing [74].
A characteristic of canines is their olfactory ability to distinguish between VOCs of different chemical nature. Lazarowski et al. investigated the olfactory capacity of retriever Labradors to differentiate between distinct nitrate salts, or ammonium-based compounds, concerning ammonium nitrate [75]. Their study reported that the ability of canines to detect other compounds (sodium nitrate and ammonium sulfate), and ammonium nitrate was 50%. This suggests that other substances used in the study differ in its smell when compared to the characteristic for ammonium nitrate.
Studies carried out in 2016 demonstrated the detection capacity of 12 anti-explosive canines trained with samples made of ANFO and its components (ammonium nitrate and fossil fuel) in different containers. In the test, 75% of the canines gave a positive response to the containers with ANFO and also for the ANFO components present in the same bin. Likewise, only 25% of the total canines gave a positive response for ammonium nitrate alone, and 33% of all evaluated canines showed interest in the containers with fossil fuel. But they did not show any warning signal that could be translated as a positive response. In conclusion, the authors reported that most canines located the smell product of the ammonium-fuel nitrate mixture and not that of the components separately [44].
Canine detection studies have shown that the training with substances responsible for positive APL detection improves the effectiveness of both training and detection. So far, only one study related to the chromatographic profile and, therefore, the identification of the VOCs released by the ANFO explosives in Colombia has been reported [49]. Despite the progress presented in this study, the chemical nature of the volatiles responsible for the positive detection by APLs manufactured with ANFO is not known yet. This lack of characterization has delayed the progress in the development of sensors that allow detecting these explosive devices with equal or higher selectivity to the presented by trained canines.
Conclusions
This review shows the current chemical strategies used in the detection on APLs, the advances reported to date for the detection of explosives, the methodologies applied to the detection of APLs, and the unknowns that still exist on the canine detection mechanism. Although there is a wide range of modern technologies used to detect explosives or APLs, canines with anti-explosive training continue to maintain a significant advantage in detection under environmental conditions. Likewise, it can be concluded that most investigations in Colombia have been focused on improving the capabilities of metal detectors. Using electromagnetic induction sensors with ground penetration radar, nuclear quadrupole resonance, or laser-induced breakdown spectroscopy to support the demining work carried out in the country.
As this works shows, there are many reported works on APL detection and very few specifically on ANFO-based APL detection, which may be because countries with high scientific research rates do not regularly suffer from attacks with ANFO-type explosives. This work shows that sensors based on nanostructured materials are the most attractive devices to develop an efficient ANFO-based APL sensor according to the topography, environmental conditions, and type of APL found in Colombia. This due to the results at the laboratory level can simply be transferred to their field operation, with easy portability, low cost, high physical-chemical stability, and with high sensitivity and reusability.
It is necessary to deepen into studies related to the search for the olfactory mark of homemade ANFO, as well as the variables that may affect the detection of this type of APL (e.g., time of manufacture and type of fossil fuel used). Indisputably, the development of a sensor with nanostructured materials requires the olfactory mark for ANFO to be fully characterized and validated by trained anti-explosive canines.