1. Introduction
Wireless Sensor Networks (WSNs) are widely deployed for monitoring purposes such as environmental and infrastructure monitoring. Typically, a WSN is composed of a large number of tiny Sensor Nodes (SNs), which are commonly battery powered and have limited energy resources. In order to save energy and to extend the lifetime of the SNs to several years, sophisticated power saving techniques must be deployed [1]. Thereby, a considerable amount of energy can be saved, while a high monitoring quality is maintained if the behavior of the SNs can be adapted dynamically to the current conditions of the system. For example, if no activity takes place in the environment, the SNs could go into sleep mode. If only spare events take place, then SNs may run a low-duty-cycling (LDC) protocol, while in phases of high and critical activity in the surrounding, the SNs should be very reactive to forward data with very low latency. Such context-aware adaptivity can be achieved in several ways, in which the transceivers and the transmission protocols play a crucial role in implementing this adaptivity and conserving energy [2].
MultiModal Wireless Sensor Networks (M2WSNs) allow using different types of transceivers and running MultiModal Switching Mechanisms (M2SMs) [3] between different protocols for reporting, depending on the current context of the environment. For instance, in some regions with high activity, the SNs start to generate sensed data that must be transmitted to the sink. Other SNs, outside of these regions, are not aware of the high activity, and they become aware of this situation by the high amount of transmissions they have to forward to the sink or by receiving an assisting message transmitted by a working node in the region [3].
Our approach considers M2WSNs where SNs have the main radio and an additional Wake-up Radio Receiver (WuRx), supporting different types of protocols. For example, all SNs can sleep until an event occurs, and when that happens, SNs are woken up by the WuRx for reporting the event to the sink. However, also all classical known LDC protocols may be executed with the main radio during some periods of activity. Thereby, we focus on the question, if there are some periods of activity, when it is beneficial regarding energy consumption to run traditional LDC protocols, instead of a wake-up protocol always using the WuRx. Consequently, the multimodality in the network is achieved by using two different transceivers and different protocols.
In this paper, we present a parameterized energy estimation model that allows us to model the behavior of particular SNs and the whole M2WSNs. We consider that this model serves as a point of comparison between wake-up protocols based on WuRx and LDC schemes regarding the power consumption. We show that WuRx with addressing will not significantly save energy compared to WuRx without addressing and that in some situations, an LDC scheme outperforms a WuRx scheme, while in some other situations, it is the other way around, giving a strong motivation for using multi-modal approaches in WSNs. Therefore, the main contribution of our work is summarized as follows: An energy consumption estimation model for MultiModal Wireless Sensor Networks that considers the behavior and performance of wake-up protocols, mainly, those based on Wake-up radio receivers. When compared to our previous work [4], this paper presents: (i) a detailed description of the proposed dual-radio layered architecture and multi-hop communications model based on WuRx for M2WSNs, (ii) an in-depth analysis of extensive simulation results that validates our proposal, and (iii) an approach to packet error and false wake-up effects in the model.
The rest of the paper is organized as follows. In Section 2, we discuss related work regarding wake-up radio and existing energy models based on WuRx, showing that there is a significant lack of models that allow comparing the energy efficiency of WSNs with WuRx and WSNs running “classical” LDC protocols. In Section 3, the models for the proposed wake-up protocol based on WuRx and LDC schemes are introduced. Then, Section 4 presents and discusses the results under various use cases, i.e., the parameter settings for the model. Finally, we conclude the paper and propose some future work in Section 5.
2. Related work
Traditional MAC protocols for WSNs are based on low-duty-cycling approaches, where SNs switch between sleep and active states, and vice versa, following a predefined or on-demand scheduling [5]. LDC approaches help reducing the energy consumed by overhearing and idle listening. However, this reduction is insufficient for low-power demand WSN designs [6], where SNs are required to save energy as much as possible to extend the network’s lifetime. Therefore, a Wake-up Radio Receiver (WuRx) approach has been proposed recently to overcome LDC limitations [1].
A WuRx is an ultra-low-power radio hardware, which is commonly added to SNs as a second radio, as shown in Figure 1. Some WuR prototypes are detailed in [2]. Typically, a WuRx is periodically listening to the channel for a pre-defined Wake-up Signal (WuS), which activates, through an interrupt signal, other electronic parts of the SNs, for instance, the main micro-controller. The wake-up receiver can be listening for WuS in the same frequency band or at different frequencies of the main radio transceiver. The former is know as in-band channel, and the latter, out-of-band channel [2]. The in-band channel is cheaper because we can use the same main radio transceiver to transmit the WuS but at the cost of dealing with interference within the neighborhood that works at the same band. The out-of-band channel approach may decrease the interference issue, but it usually adds complexity and extra cost to the system design (two radio, one for WuS transmission, and another for data communication). However, compared to “classical” low-power radios, the power consumption of a WuRx is several orders of magnitude lower than these radios, allowing to keep it always-on [2]. Consequently, a WuRx can eliminate the idle-listening and waiting time issues, and help reduce the energy consumption and latency [1].
Some WuRx implementations have dedicated circuitry to perform an addressing mechanism by decoding a destination address contained in the packet header. Therefore, only the designate node is woken up instead of the entire neighborhood. This feature might allow solving the overhearing issue presented in LDC approaches. In this sense, there are mainly two manners to consider the recipient of a WuS. On the one hand, a source node can reach all the SNs within its neighborhood by a broadcast-based wake-up. All SNs within the source range receive the WuS. On the order hand, a source node intends to reach only one node within its range using dedicated circuitry. This scheme is usually known as ID-based scheme [2]. The latter is intended for selective wake-up addressing, where only the node with a specific ID is woken up. This scheme helps to reduce the false-wake-ups and the overall energy consumption of the whole M2WSNs (usually in large-scale deployments), but it requires a decoding process which is usually performed by an external micro-controller that adds an additional energy requirement to the WuRx power supply. Besides, the WuS packet needs extras bits (e.g., 2 bits [2]) for the destination address, that might require extra transmit time, hence extra energy consumption. The former can contribute to reduce the end-to-end latency, because the node does not decode the incoming WuS, but it might increase the false-wake-ups, which may be potentially costly regarding energy consumption. Therefore, there is a trade-off to be made between latency and false-wake-ups reduction.
Piyare et al. [2] made a comprehensive overview of WuRx based on MAC protocols and offered an extended taxonomy of WuRx based on routing protocols compare to Djiroun and Djenouri’s work [1]. They concluded that the lifetime of the SNs could be further extended by combining WuRx capabilities with selective addressing and routing duties (e.g., T-ROME [5]) while meeting latency requirements comparable to SNs that use a single radio.
In Table 1, a comparison is made between relevant energy consumption models proposed in the literature and our approach. Most of the work only applies for single-hop MAC protocols and two-channel solutions. Our model takes into account multi-hop communications and the energy budget for the whole network, and the main radio and WuRx use the same channel (in-band-solution), whereby only very few works exist in that area [2]. Finally, our model allows for modeling addressing and non-addressing WuRx compared to other models that focus only on addressing.
3. Energy consumption models
Typically, the wake-up protocol is integrated into the link layer of a layered architecture for M2WSNs [Figure 2], which includes an application layer which runs M2SMs (e.g., eHNS [3]) using the information provided by its adjacent layer. A network layer that performs topology management duties, e.g., path selection, and packet forwarding. A link layer that executes sleep-wakeup duties combined to medium access control with retransmission functions, which aim to minimize the energy consumption at the physical layer by reducing the transmission power while providing a high monitoring quality. In this paper, we focus only on the lower-layers and consider a dual radio communication (main radio and WuRx) at the physical layer, both radios sharing the same channel (in-band solution).
Figure 3 shows an example of a multi-hop communications on a tree-like topology for reporting an event and its associated data to the sink using a dual radio. A source SN detects an event, reports and propagates it through a known routing path towards the sink. In this schematic, the WuRx range is shorter than the main radio range. The routing path is constructed and updated by a “classical” routing protocol (e.g., RIME or RPL as done in [11]) at the network layer. Finally, the source SNs, before transmitting its sensed data towards the sink, sends a WuS packet, which wakes up all potential receivers (i.e., child and parents SNs) within the wake-up radio range (i.e., those SNs that have a WuRx integrated), as shown in the right sketch on Figure 3. Later in this paper, we give more details about this operation.
The state machine diagram for such a dual radio setup of a single node is shown in Figure 4. The dashed rectangles are transition states, and the power consumption of a state, , and the period a node remains in each state,
, are shown in round brackets. The WuRx has one main state, channel − listening, and a transition state, WuRx − Setup, that models its initial setup. After the setting up state, the WuRx remains listening to the channel, waiting for a WuS to arrive. Upon a WuS, the node is woken up by an external interrupt signal generated from the WuRx, as shown in Figure 1. In this case, the main radio of the node stays in its deepest sleep mode (DLPM) with the lowest power consumption PDLPM. Otherwise, if the SN runs a LDC protocol without WuRx-support, some timers are needed to wake the SN up periodically (the SN stays only in a low-power mode (LPM) with power consumption PLPM), and typically, PLPM is larger than PDLPM.
During the active mode, the main radio remains on its Idle state, and switches between Transmitting and Receiving states depending on the task to perform: (1) to transmit a data or Ack packet to the next hop in the routing path or (2) to receive a data or Ack packet from the previous hop (to process it or to relay it). The transitions to set up the main radio are modeled in Tx Setup and Rx Setup states (e.g., data encapsulation), respectively. After performing these tasks, the main radio returns to its Idle state, and then to the Sleeping state, where it remains in the deepest low power mode.
In the subsequent sections, we present our underlying assumptions and briefly describe the protocol schemes for both cases, i.e., using the wake-up protocol based on the WuRx and based on the LDC scheme. Based on these descriptions, the energy model for delivering a packet over a single path to a sink is presented, which resembles already known models. Finally, these models are extended to cover the energy consumption of the whole network, based on the node density and event rates.
3.1 Basic Assumptions
In our analytic model and to compare the benefits of a WuRx and a LDC configuration for M2WSNs, some underlying assumptions are made:
The WuRx and the main radio share both the same channel. As a consequence, transmissions of the main radio are detected by the WuRx. Therefore, each SN needs, besides the main radio, only a WuRx, and not a wake-up transmitter.
Both radios have the same communication range.
We do not care about the network topology and routing protocols. We assume that a SN knows the address of the next hop for delivering a data packet to the sink.
Transmitted data message is very small. Therefore, we assume that it is enclosed in the wake-up packet.
For the sake of simplicity, we assume that the power consumption (Pnode) of the main radio is the same for the wake-up and idle periods, receiving and transmitting a packet, and switching between states.
For energy estimation, we assume no packet loss during communication, i.e., an error-free cannel [Table 1].
All SNs sleep in LPM4 mode, i.e., the deepest operating sleep mode, until they are woken up for their active period.
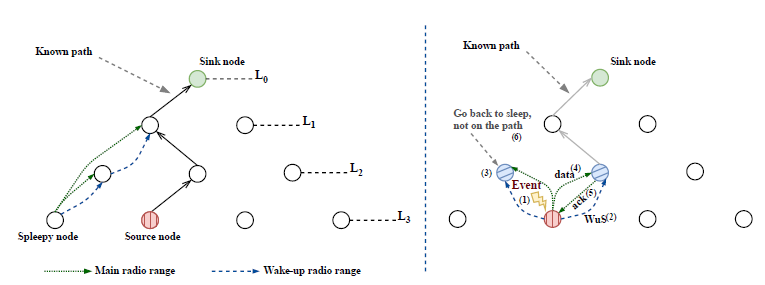
Figure 3 A dual radio multi-hop communication schematic on a tree-like topology (Based on [5]). The right sketch shows a multi-hop operation of the wake-up protocol proposed. The event data packet is propagated from the source to the sink through a known routing path previously defined
3.2 Wake-up protocol based on a WuRx scheme
Figure 5 presents the behavior of the proposed wake-up protocol using a WuRx scheme when delivering a data packet to the sink through a known routing path. The diagram shows the packets transmitted using the main radio. In Table 2, we also give a list of variables and their corresponding description, which are used in the diagrams and the later analysis.
The timing diagram in Figure 5 presents the interactions between the SNs within the established path to the sink. The wake-up protocol operates under a transmitter-initiator scheme, where the source node (i.e., the sender node) or working node [3] starts the communication by first sending a WuS packet (a dummy ack packet) using its main radio and then data packets with destination address. After sending a data packet, the sender waits for an acknowledge packet (ack) (e.g., ), and if no ack-packet is received, the sender transmits the same data packet again, until an ack-packet arrives or the number of trials is exhausted. The WuS-packet wakes up all potential receivers, i.e., those SNs within the interference range of the sender node (as shown in Figure 3, step 3). Hence, also the non-destination SNs turn on their main radio, remain active until a data packet is received, but then go back to sleep because the destination address does not match their own address (refer to step 6 in Figure 3]. For simplicity in Figure 5, the non-destination SNs interactions are not shown.
The designated receiver (i.e., Hop − 1, the node within the multi-hop routing path) should receive one of the subsequent data packets, and then, send an ack-packet back to the sender. This ack-packet also serves as a WuS-packet which wakes up the next hop (i.e., Hop − 2), and Hop−1 can forward the data packet toHop−2, right after sending the ack packet. Finally, Hop − 1 goes back to sleep. This procedure is repeated throughout the hops in the known path until the data packet is delivered to the sink, as shown in Figure 5.
Energy model for a single path
Based on the assumptions made and the timing diagram of Figure 5, the energy budget, Ehop in equation (1), allocated to a single intermediate node (e.g., Hop − 1) on a known path is given by:
where PΔDLPM = Pnode − PDLPM is the power consumed in active mode on top of the power consumed in DLPM.
Consequently, the energy budget allocated for transmitting a single data packet over h many hops on the known path is given by the equation (2).
The first term gives the power consumption of the h − 1 intermediate SNs on the path, while the second term complements the power consumption of the source and sink, Esource−sink in equation (3), which it is detailed below:
Depending on the WuRx used (supporting addressing or non-addressing), also other SNs in the surrounding of the SNs on the path are woken up. In our model, we denote this number of nodes as nbor and assume that in M2WSNs with equally distributed SNs, nbor is constant for every node. If the WuRx supports addressing, then nbor = 1, otherwise, we assume that each node on the path wakes up nbor > 1 SNs in its surrounding. These non-destination SNs stay awake until they have received a data packet with the destination address. Afterward, SNs realize that they are not the destination and can go back to sleep. Hence, the energy consumed by these SNs during their active period, Enon−dst, states in equation (4).
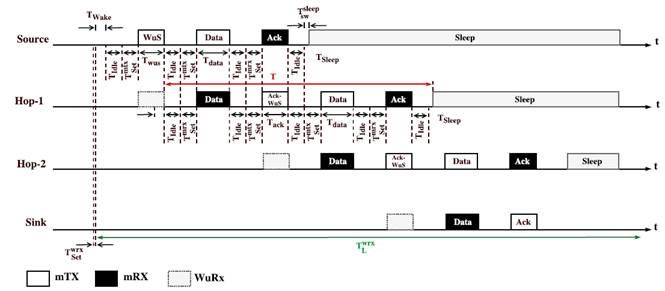
Figure 5 Timing diagram for delivering one event data packet over hops through the known path Based on [8]) using a WuRx scheme. The non-destination SNs (not within the known path) interactions are not shown in the diagram
Therefore, the total energy consumed by each event to deliver it towards the sink (only if a wake-up event occurs), ewakeupevent , considering equations (2) and (4), is given by the equation (5).
Energy model for the complete M2WSNs
Now, we provide the model for the energy consumption of the whole M2WSNs. We divide the total energy consumption of the M2WSNs in two parts, as shown in equation (6).
The first term covers the base-energy consumption that is always present in DLPM. ebase is the power consumed in the DLPM by each node over time and its WuRx, during the total runtime of the M2WSNs, and it is defined as shown in equation (7).
The second term in equation (6) covers the energy consumption on top of the base-energy-consumption during the active period of SNs. Depending on the activity in the network, the energy budget for all events is given by the equation (8).
where trun/mtbe is the number of events during the total runtime (trun).
3.3 Wake-up protocol based on a LDC scheme
The wake-up protocol presented in this section resembles the ideas of already existing protocols based on LDC schemes such as those proposed in [13]. The protocol follows the radio state machine introduced in Figure 4, without the states related to the WuRx, a transition between Sleeping and Wake-up states is added (which occurs after a timer expired), and use LPM instead of DLPM. Hence, the single radio state machine for LDC schemes results as shown in Figure 6.
The wake-up protocol operates under a sender-initiated LDC scheme. Every node periodically wakes up to listen to the communication channel, and to determine if there are potential incoming data packets during a TAwake period. If no data packet is detected, the node goes back to LPM and sleeps until its next scheduled wake-up interval (e.g., TSleep), as shown in Figure 7 (i.e., refer to Hop − 1 time line).
When a sender node has a data packet to transmit, it repeatedly sends a beacon to its neighbors until an ack-packet is received (every TIdle + Pmtx Set). The beacon is the full data packet with the destination address (useful if a payload is small). Therefore, only the designated receiver (e.g., Hop − 1) acknowledges the received data packet. After receiving the ack packet, the sender node stops transmitting the data packet and goes back to the sleep period, as shown in Figure 7. This procedure is followed by each hop until the data packet is delivered to the sink.
Energy model for a single path
By following an approach similar to that of Section 3.2, we came up with the energy budget allocated for delivering an event data packet to the sink over h many hops as states equation (9).
where ehop = erecv + efwd is the energy budget allocated to a node to forward an event to the next hop. This energy budget is composed of two parts. The first part, erecv, covers the energy for receiving the packet, and is given by the equation (10).
Receiving takes place during the regular listen period of a node, whose energy budget is already covered by ebase in (12). For that reason, no additional energy budget needs to be allocated, except the energy to receive the ack-packet. The second part, efwd, gives the energy budget required to forward the packet, which takes place usually during the regular sleep phase of receiver SNs. In the worst-case, the sender hits the active-period of the receiver after time Tsleep+2Tdata. In the best case, the first data packet of the sender hits the active-period of the receiver. We assume that the active period of the receiver is hit on average after half the worst-case time. Hence, efwd is given by the equation (11).
Energy model for the whole M2WSNs
The total energy consumed by the whole M2WSNs is also composed by two part as states in (6), but the formulas for e base and e active are given by equations (12) and (13).
where T on and T off are the sum of the active and sleep times, respectively, of all SNs, as shown in equation (14).
where T = T
Wake
+ T
Awake
+ + T
Sleep
is the duration of a whole wakeup-cycle as shown in Figure 7. The ebase in (12) summarizes the energy budget needed for the regular wake-up and sleep cycles, i.e., if no transmission occurs at all in the M2WSNs, then only e
base
is consumed. Meanwhile, the e
active
in (13) is the energy budget allocated to the transmission of a single event to the sink. This energy budget includes only the additional energy needed on top of e
base
for transmitting the event to the sink.
4. Results
Based on the proposed energy model (6), we can estimate the total power consumption for the whole M2WSNs using both schemes under different WSN configurations varying the number of SNs (N), event frequency (mtbe), number of hops in the known path (h), and the number of woken up neighbors along the path (nbor). Thus, we perform a parameter sensitivity study for different configurations (i.e., the parameter settings for the model). Table 3 shows the values employed in the models, which are based On a mote constructed with the commercial micro-controller MSP430 and transceiver C1101 at 868 MHz along with a WuRx. This WuRx allows for permanently listening while consuming 150μW.
First, we analyzed the impact on the energy consumption when considering different low-power modes supported by the node employing the WuRx, as shown in Table 4. For the first scenario, we considered the classical use case where P wrx +P DLPM < P LPM , with P DLPM = 0.1μA×3.3. V nine times lower than P LPM = 9 × P DLPM (assuming that all peripherals can be turned off). For this scenario, varying mtbe and T Sleep shows that a significant energy budget could be saved when this WuRx protocol is used, i.e., the ratio between WuRx and LDC power consumption ranges from 0.02 to 0.05.
In the second scenario, we used the real P wrx value, where P wurx + P DLPM > P LPM . Although the power consumption of the SN using the WuRx is higher than without WuRx, using the WuRx protocol still saves 50% of the energy budget. However, the energy consumption is very sensitive to the traffic load, as shown in Table 4, where the LDC scheme becomes better than WuRx scheme for long sleep periods and low event rates. Hence in such situations, the LDC scheme should be used instead.
In the third scenario, we assumed P DLPM = P LPM = 6.6mA × 3.3.V, reflecting the case that the WuRx configuration cannot benefit on the SN from a DLPM, e.g., due to some peripherals that must be turned on all the time. If we employ the same LPM, both wake-up protocols cause almost the same energy consumption, being WuRx scheme a little better than LDC scheme. This scenario is also less sensitive to the network activity.
In sum, a dual radio communication becomes beneficial in scenarios of short and long sleep periods, and light and heavy event rates, when the energy consumption of the WuRx is in the order of some micro-watts, and the SN remains in its deepest low power mode (i.e., LPM4). For P wurx + P DLPM ≥ P LPM , the wake-up protocol based on WuRx performs better than LDC schemes in circumstances of high event occurrence rates, due to its always-on listening mode.
The curves in Figure 8 are obtained by varying the same single parameter in both models. Besides, the study is made under the third scenario, where mtbe = 60s and T sleep = 10s. For each parameter value, the power consumption in each model was computed, and the ratio, i.e., power consumption WuRx-based model/power consumption LDC-based model, is shown on the y −axis. Hence, if the ratio is less than one, the power consumption in the WuRx-based model is smaller than the LDC-based model, and vice-versa.
The effect of varying the nbor is negligible, according to the results presented in the top left graphic. When nbor = 1 (i.e., addressing in WuRx is used) the energy consumption is almost the same as for larger nbor values (modeling no addressing). We can conclude that a broadcast-based wake-up is not energy costly compared to an ID-based wake-up when the size of the neighborhood increases and a high event rate traffic is considered. Besides, compared to a WuRx that supports a WuS decoding operation, the total power consumed for waking up the microcontroller using an interruption signal, which in turn switches on the main radio transceiver to process the incoming data packet, and then, to go back to the sleep mode, is insignificant.
Increasing the number of SNs in the M2WSN (N) has little effect, as shown in the top right graph, which implies that the total size of the M2WSN does not affect the effectiveness of a particular protocol scheme, if the other parameters are constant. However, the performance of the wake-up protocol based on WuRx decreases as N increases, due to the energy consumed by non-destination SNs that remain awake when an event occurred and until they realize that they are not the destination node. In this situation, it might be beneficial to use a WuRx with addressing support and a false wake-ups reduction strategy.
For higher number of hops (h) (third bottom left graph on Figure 8], the protocol based on WuRx performs better than based on LDC and becomes beneficial, thanks to the WuRx operations that allow SNs within the routing path to remain less time waiting for the next hop to wake up compared to LDC schemes.
Finally, for short event periods (mtbe), WuRx scheme is better, but somewhat affected by long event periods, due to the always-on operation assumed for the WuRx.
5. Conclusions and future work
In this paper, we presented an energy consumption estimation model that considers the behavior and performance of wake-up protocols based on Wake-up Radio Receivers (WuRx) and the traditional low-duty-cycling (LDC) schemes employed in M2WSNs and multi-hop communications.
M2WSNs based on an always-on ultra-low-power WuRx schemes save significantly more energy compared to “classic” LDC schemes when using many hops on the routing path and for short event periods. Besides, the WuRx with addressing does not significantly save energy compared to WuRx without addressing, under a traffic load with low event rates. However, for circumstances of long event rates, the WuRx scheme might lose against an LDC approach regarding idle-listening. Duty-cycling the WuRx could be considered as a possible solution to this issue.
In the energy consumption model based on WuRx, we considered the worst case, i.e., where all SNs within a neighborhood into the M2WSNs are woken-up when a source node has an event packet to transmit towards the sink. Therefore, the energy consumed due to false wake-up is implicitly considered in the model, i.e., equation (4). The expectation of successful event-packet transmitted to the sink can be modeled as a factor, which follows a distribution probability. This factor can be introduced into equation (2).
Therefore, as future work, we propose to include the effect of packet error losses into the model. Besides, model validation is required under emulations and laboratory-based experiments. In addition, we expect to incorporate the results into a multimodal switching scheme to dynamically select the appropriate wake-up protocol based on the circumstance, getting the best of both approaches (i.e., WuRx and LDC).