1. Introduction
Metals are naturally redistributed in the environment by geological and biological cycles. Among the biological cycles of movement of metals are the processes of biomagnification and bioaccumulation. These phenomena happen when a substance is present in low concentrations at the beginning of the food chain and increases in proportion as it moves up the food chain[1, 2]. Metals can be distributed in the environment due to anthropogenic activities, which shorten their residence time in mineral deposits and result in the formation of new metal compounds of unnatural origin[3, 4]. The industry mostly performs a global distribution of metals in the environment by discharge to land, water and air[5].
Lead is used in different types of industries, in the petrochemical industry, it is employed to make batteries, pigments and paints, and in agriculture, it is utilised to make fertilisers[6, 7]. Additionally, Wastewater effluents from processes such as electroplating, metal finishing, metallurgy, chemical manufacturing and battery manufacturing industries contain substances with the chemical species Ni0 or Ni2+[8]. These chemicals could cause cyanosis, chest pain, dry cough, extreme weakness and dizziness, rapid breathing, severe headache, illness, shortness of breath and vomiting, and in high concentrations are carcinogenic[9-11]. After the metals are used, they end up as part of the effluent from the process in which they were used, which generally receives treatment that is often inefficient and in the worst-case non-existent, generating serious contamination problems when they are discharged into water sources[12]. Thus, there is a great need for a simple, effective, and low-cost method of treatment for the disposal of metals in wastewater[13, 14]. In this sense, adsorption processes are presented as an alternative, since when combined with an appropriate desorption step and avoiding the problem of adsorbent removal, they are an effective, versatile, and low-cost method that makes it sustainable for the removal of contaminants. However, commercial adsorbents tend to be expensive, so new economical adsorbents with high removal capacity have been examined[15].
Different lignocellulosic biomasses have been used in the removal of Pb (II) and Ni (II), such as millet[16], lime[9, 17], rice husks[16, 18], black walnut bark[19], among others[20-24]; presenting high removal yields. The objective of the present study is to use the lemon peel as a bio-adsorbent of Pb(II) and Ni(II) in aqueous solution in selective and multicomponent systems, in the latter case, since both for industrial discharges and wastewater discharges the pollutants are mixed. The interaction between these pollutants should be studied, evaluating the effect of temperature, adsorbent dose, and particle size on the process.
2. Methodology
2.1 Materials
The lemon peels were collected from a commercial market in Cartagena, Bolivar. Silver nitrate (Pb(NO3)2) and nickel sulphate (NiSO₄), Sigma Aldrich brand analytical grade, were used to prepare the solutions. Sodium Hydroxide (NaOH) and Hydrochloric Acid were used to adjust the pH of the solutions.
2.2 Preparation and characterization of biomass
The agricultural residues were washed to remove impurities, sun-dried to a constant mass, and the particle size was reduced (0.355, 0.677 and 1 mm).
Then, the biomaterial was characterized by Fourier Transform Infrared Spectroscopy analysis in a Shimadzu IRAinfinity-1S spectrophotometer with a frequency of 32 scans between 400-4,000 cm-1, in the purpose of identifying the functional groups that would work as active centres during the process of metal removal. The morphological properties and elemental composition of the biomass were determined using a Scanning Electron Microscope together with an energy dispersive spectroscopy (SEM-EDS) model JSM-6490LV JEOL Ltd. All trials were conducted before and after the metal removal process.
2.3 Adsorption study
Adsorption tests were performed following a continuous linear factor experiment design, on a central composite response surface: Star, considering a randomized central point for each established range provided by the Statgraphics Centurion 16.1.15 software. The response variable was the percentage of removal, and the independent variables were particle size (0.355, 0.677 and 1 mm), temperature (40, 55, 70 ºC) and adsorbent concentration (0.15, 0.325 and 0.5 g) at 200 rpm, and pH 5 for Lead (II) and 6 for Nickel (II). An orbital shaker, MAXQ 4450, was used to place the contaminated solution in contact with the adsorbent under the different conditions established in the design of the experiments. The residual concentration was determined by atomic absorption at 217 nm for Pb(II) and 232 nm for Ni(II). Adsorption efficiency and capacity were determined by Equations (1) and (2), where C 0 is the initial contaminant concentration and C f the final concentration in mg/L, V the volume in L, m the amount of adsorbent, %R the removal efficiency and q the adsorption capacity of the bio-adsorbent.
2.4 Kinetic study and modelling of balance
The kinetic study was carried out under the best conditions found after applying the Response Surface Methodology (RSM), taking 8 samples at different intervals during 24 h. For this, 10 mL of solution at 0.5 mmol/L at 200 rpm was placed in contact. The data were then fitted to the kinetic models (Pseudo-First Order, Pseudo-Second Order, Elovich and Intraparticle Diffusion) using OriginPro 8.5 to calculate the fitting parameters and R2. Adsorption equilibrium was performed for 24 h by varying the initial concentration of contaminant (20, 40, 60 and 80 mg/L for Pb (II) and 5, 10, 15 and 20 mg/L for Ni (II). Langmuir and Freundlich's models were used to adjust the data.
2.5 Thermodynamic parameters
The adsorption capacity of the adsorbent Qt, the equilibrium constant Kc and the values of adsorption enthalpy ∆Hº, adsorption entropy ∆Sº and Gibbs energy ∆Gº were calculated to establish the favourability of the process and the effect that temperature has on it. For this purpose, temperature values corresponding to a typical adsorbent dose were selected, the final ion concentration was tabulated for each of the different temperatures, and the parameters were calculated according to Equations (3), (4), (5) and (6), applying Van't Hoff's graphic method.
Where, Kc is the equilibrium constant, qe is the equilibrium solid phase concentration (mg/g), and Ce is the equilibrium concentration (mg/g), R is the ideal gas constant 8.314 J/molK, T is the absolute temperature in K. The ΔH° and ΔS° are determined from the slope and the Y-axis intercept of Arrhenius of lnKc vs T -1 , respectively.
3. Results
3.1 Characterization techniques
The process of adsorption is a physicochemical phenomenon that involves many steps and mechanisms, consequently many factors can intervene during ion exchange between the bio-adsorbent and sorbate. Thus, the presence of functional groups involved in the adsorption was determined by Fourier Transformed Infrared Spectroscopy (FTIR) and are shown in Figure 1, confirming the complexity of lignocellulosic materials[23].
A peak at 3,314.61 cm-1 is observed, shown in the spectrum before the adsorption process, which is an indication of the stretching of hydroxyl groups in the molecular structure of the lemon peel[25, 26]. Also, vibrations are observed at 3,390.28 cm-1 (ternary amines), 2,931.01 cm-1 (methoxy and aliphatic hydrocarbons), 1,652.11 cm-1 (C=C), 1,420.81 cm-1 (C-C), 1,339.79 cm-1 (NO2), and 1,156.40 cm-1 (C-O). After the removal of Pb(II) and Ni(II), a spike shift and widening are observed, which can be attributed to the bonding of the metal ions with the identified active centres[27].
Figure 2 shows the SEM microphotography of the lemon peel before and after the removal of Pb(II) and Ni(II). The surface of the biomaterial is irregular, and porous allowing a better heterogeneous biosorption due to the large interface [Figure 2a); moreover, it presents small white spots which are caused by the presence of Calcium, Aluminium and Potassium in its structure [Figure 3a). The SEM micrographs after adsorption [Figures 2b and 2c), show Pb(II) and Ni(II) agglomerations on their surface, which can be attributed to the formation of chelates in the samples[28]. This fact is confirmed in the EDS analysis after metal removal, Figures 3b and 3c, which show the appearance of the characteristic high-intensity peaks for Pb and Ni at 1.7 and 0.8 keV, respectively. This event was due to the mechanisms that appear during the adsorption process of metals on bio-adsorbents, which include ion exchange, microprecipitation, complexation and coordination, due to the presence of functional groups on the surface of the adsorbent[29, 30].
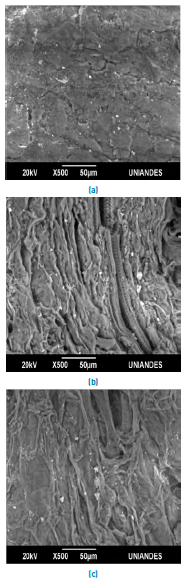
Figure 2 SEM micrographs of lemon peel (a) unsaturated and after adsorption of, (b) Pb (II) and (c) Ni (II)
Figure 3 shows the spectrogram and elemental composition of the selected biomasses obtained by EDS analysis after the Pb(II) and Ni(II) adsorption process. Figure 3a revealed that carbon and oxygen contribute more to the elemental composition of the biomass, which is attributed to the organic nature of the lignocellulosic residues[27], and its ability to capture cations by electrostatic forces[31]. The presence of both heavy metals on the adsorption surface was evidenced with a mass percentage of 0.26 and 0.11%, respectively. After removal of Pb (II), the disappearance of aluminium was evidenced, this was found in 0.24% in the structure of lemon peel. In the same way, the decrease of calcium and potassium [Figures 3b and 3c).
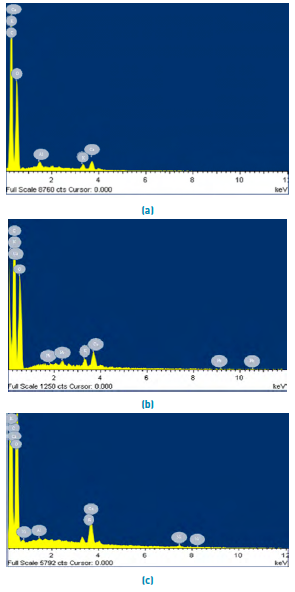
Figure 3 EDS spectrum of lemon peel (a) unsaturated and after adsorption of, (b) Pb (II) and (c) Ni (II)
These results can be attributed to the formation of links between the active sites of materials and lignocellulosic ions[32]. Also, there was a decrease in the presence of C in the biomaterial. This fact was due to the saturation of active sites at sampling time. In EDS spectra, after the adsorption of Pb (II) the disappearance of Al is observed at 1.8 keV, and of Ca at 0.6 keV. On the other hand, after removing Ni (II), there was an increase in K in 0.2 and 3.4 and Ca growth in 3.4 keV. From the previous behaviour, a conservation in the variation of the equivalent charges was found between Ni (II), Pb (II) and Ca (II) / K, so that the ions present more competition understudy for the functional groups whereby cation exchange occurs in the active centres of the bio adsorbent. In the same way, the formation of micro-complexes and micro-precipitations on the surface of the lemon peel could take place[30]. The increase in the atomic percentage of Ca and K after Ni (II) adsorption shows that these elements precipitate in calcium and potassium phosphate and phosphate forms on the surface of the biomaterial, which is seen in the white particles in SEM micrographs[33, 34].
3.2 Particle size effect
The particle size at adsorption is a determining parameter, as it is related to the surface contact area of the bio-adsorbent and the availability of active sites[35]. In Figure 4, the effect of particle size for Pb(II) and Ni(II) using lemon peel is shown. It was found that the highest adsorption efficiencies were achieved using 1 mm size, with 95.33% lead adsorption performance and a final concentration of 4.66 mg/L, and 62.133 %, for 0.355 mm size nickel with a final concentration of 37.86 mg/L.
Adsorption takes place mainly inside the particles, on the pore walls at specific points. Therefore the amount of adsorbate (solute) that can be adsorbed is directly proportional to the volume of the pores, and this volume is directly proportional to the external contact area[36]. The results shown in Figure 1 may indicate the possibility that the adsorption of the metal ions occurs inside the particles through the porosities of the biomaterial. Also, a small particle has a larger surface area, thus increasing the number of pores per unit mass[37]. In other words, when the size of the particles decreases, the number of active centres per unit is higher, and the adsorption capacity increases[38]. Likewise, the nature of the pollutant and the selectivity of the active sites are determining factors during the adsorption processes[39].
3.3 Effect of temperature and dose of adsorbent
The dosage study is essential in adsorption experiments to determine the proportion between the mass of the adsorbent and the initial metal concentration. The removal efficiency is highly dependent on the type and amount of adsorbent, so a dose increment generally increases the amount of ion adsorbed due to the larger area available for the process[40]. On the other hand, the temperature is a parameter that provides information about nature and forces that control the process[41]. Figure 5 shows the Pareto diagram for Pb(II) and Ni(II) adsorption. It was observed that temperature significantly affects the removal process in a negative way, while the dose of adsorbent is the most influential variable in the process.
In Table 1, the results obtained from the analysis of variance with a 95% confidence level are shown, finding that, 0 effects have a P-value lower than 0.05, indicating that they are significantly different from zero with a 95.0% confidence level. It is observed that the factor that may have greater relevance in the design is the amount of adsorbent (g).
Figures 6 and 7 show the variation in the percentage of adsorption concerning temperature and the dose of the adsorbent for Ni(II) and Pb(II), respectively. The decrease in the percentage of Ni (II) removal is proportional to the increase in temperature, which suggests that this process is exothermic, and it does not need an energy supply to the system. In this way, it would increase the kinetic energy of the ions weakening the interaction forces between adsorbate and adsorbent, and favouring the desorption of the sorbate molecules over the bio-sorbent structure. Since by gaining more energy than the bonds formed between the compounds support, the forces of interaction are reduced, causing the release of what is not desired; this is promoted by the temperature, decreasing the net adsorption capacity of the adsorbent[42-44].
From the adsorption tests carried out, the Response Surface Methodology (RSM) was used to determine the optimal conditions of particle size, adsorbent dose, and temperature. The optimal adsorption conditions are shown in Table 2 and will be used to perform the kinetic and equilibrium study.
3.4 Modelling of adsorption kinetics
The adsorption kinetics of an adsorbate provides information about the saturation time of the adsorbent and the maximum adsorption capacity before saturation is reached [45]. The adsorption kinetics of Pb(II) and Ni (II) on the lemon peel, respectively, are shown in Figures 8 and 9, as well as the fit to the Pseudo-First Order (PFO), Pseudo-Second Order (PSO) and Elovich models. The adjustment parameters to the kinetic models are shown in Table 3.
Based on Figures 5 and 6, it is established that the adsorption of the ions occurs rapidly in the first minutes of the process, due to the availability of active sites, the equilibrium times were 180 and 300 min for Pb (II) and Ni (II), respectively. In the case of both metals, about 70% of equilibrium concentration was reached at minute 120, indicating saturation of available sites in the adsorbent. Therefore, the capacity does not change significantly at longer times.
From the model fit, it was found that pseudo-first order and pseudo-second-order models show a better description of the experimental adsorption data for both metals. From the values of the constants k1 and k2, it is determined that the initial absorption rate for lead is lower than for Ni. It can be said that two active sites in the biomass adsorb the ions and that the process occurs by chemisorption. This fact is due to the formation of chemical bonds between adsorbent and adsorbate at the surface[46, 47]. However, the Elovich model also fits well, so it is established that adsorption takes place inside the pores of the particles of the biomaterial and that their active sites are heterogeneous, which corresponds to the FTIR spectrum of lemon peel [Figure 1], where the complexity of the material is evidenced by the presence of multiple functional groups in its structure, characteristic of its lignocellulosic nature, as well as the high presence of C and O shown in the EDS spectrum [Figure 3a); hence, they exhibit different activation energies throughout the adsorption process[48, 49].
Adjusting the adsorption kinetics of Ni (II) and Pb (II) on the lemon peel suggests that the adsorption is carried out in two steps. Firstly, the metal diffuses through the solution until it reaches the external surface of the bio-adsorbent, so there would be a rapid adsorption rate during the first minutes of the process and micro-precipitates would form in the external contact area [Figures 3b and 3c)[50, 51]. In the second one, there would be the process of diffusion of Lead and Nickel through the pores of the adsorbent material, so that the process would take place in multiple internal active sites until saturation is reached, promoting the formation of complexes. Likewise, as the graph cuts the origin, it can be said that the mechanisms that dominate the removal process are intraparticle diffusion and chemical and physical adsorption[52].
3.5 Adsorption equilibrium
The adjustment of the lead and nickel adsorption equilibrium data is shown in Figures 10 and 11, respectively. The Langmuir, Freundlich and Dubinin-Radushkevich isothermal models were used and valuated by nonlinear regression. This process establishes the driving forces governing the interaction between the adsorbate and the adsorbent and the fitting parameters are shown in Table 3.
It was found that both models adjust the Pb (II) adsorption equilibrium data satisfactorily, which indicates that both mechanisms have an incidence. However, physical forces prevail over these, and the formation of a monolayer limits the process, no desorption reaction is considered. The number of species adsorbed does not exceed the number of active site[53]. The Freundlich model predicts the process of Ni (II) adsorption, so it can be said that during the adsorption process multilayers are formed on the surface of the biomaterials with a non-uniform distribution of heat and adsorption affinities on the heterogeneous surface. The active sites of absorption are occupied first by strong bonds, and this force decreases as they are occupied by the ions[54, 55]. The value of Freundlich's constant n [Table 4], is not in the range 1-10 which indicates that the chemical bonds formed between the ions and adsorbent are weak[56, 57].
From equilibrium data to the Dubinin-Radushkevich model, a good fit was found for the Ni (II) and Pb (II) isotherm, with an R2 higher than 0.98 in both cases and a qmax close to the experimental one. The values reported for parameter E (average adsorption energy per adsorbate molecule required to transfer one mole of the ion from the solution to the adsorbent surface), suggest that the process is mostly controlled by physisorption[58]. The fit of the data to this model assumes that the lemon peel presents a heterogeneous structure, as shown in the FTIR spectrum [Figure 1] due to the presence of the multiple characteristic functional groups of the hydrocarbon lignocellulosic compounds. The Dubinin-Radushkevich isotherm requires this kind of materials since it assumes that active sites have different activation energies[58].
3.6 Thermodynamic parameters
The change in Gibbs' standard free energy (ΔG°), standard enthalpy (ΔH°) and standard entropy (ΔS°) as a result of temperature allows establishing the possible mechanisms of adsorption process (endothermic/exothermic, favourability and spontaneity of the process). The thermodynamic parameters mentioned were calculated by applying the Van't Hoff graphical method and Equations 3 to 6. Results are shown in Table 5.
The positive value of enthalpy for Pb(II) shows that the process is endothermic, while that of Ni(II) is exothermic, which is consistent with the effect graphs [Figures 3 and 4][59]. The negative values of ΔS suggest that the biomass has an affinity for the ion forming strong bonds on the surface, thus obtaining a low possibility of reversibility[59]. The positive values of Gibbs' free energy indicate that the adsorption process is not spontaneous or favourable, and the reaction is not feasible at high temperatures[35, 60].
3.7 Adsorption in binary system
Figure 12 shows the lead adsorption in the presence of Nickel (29.345 mg/L) while varying the initial lead concentration (41.4, 103.6 and 207.2 mg/L.), the results are also compared with the individual Nickel adsorption. It was found that the adsorption of these two metals is not significantly affected by the simultaneous presence of the other in solution.
However, Figure 12 shows the gradual increase in the adsorption capacity of Ni (II) in the presence of Pb (II). This is because the two metals in solution would increase the electrostatic force between the ion and the active sites of the contaminant, causing the increase in the adsorption capacity of the bio-sorbent, with Pb (II) having a higher affinity for the active sites[61]. An increase in the adsorption capacity of Ni (II) was observed from 3.69 mg/g to 4.57 mg/g, which represents an increase of 19.26%, as the concentration of Pb (II) increased from 41.1 to 207.7 mg/L. In contrast, the adsorption capacity of Pb (II) was 8.32%. This event could be because both metals compete for the active centres of the biomaterial and to a possible crosslinking of the adsorption sites so that a small amount of Ni (II) could replace Pb (II) during the adsorption process, as the concentration of the interfering metal increased[62, 63].
Figure 13 shows the adsorption of Nickel in the presence of Lead by varying the initial concentration of nickel (11.7, 29.3 and 58.6 mg/L), comparing the results for individual Lead adsorption. It was found that the behaviour for the metals is similar to that presented during the binary assays of Pb(II) in the presence of Ni(II), since the removal capacity of Pb(II) increased. Likewise, nickel obtains high adsorption, so it is noted that the lemon peel presents a significant amount of active sites to allow high adsorption of both metals, with very low interference[64]. Despite the concentration of both metals increased when found in the binarye system, the increase was lower than in the previous system, because Pb (II) concentration of only grew by 0.41% and that of Ni (II) by 18%. The lower adsorption capacity of the Ni (II) ion is related to that, the higher hydration energy of this ion compared to the Pb (II) ion (2,105.0 kJ/mol for Nickel and 1,500.6 kJ/mol for Lead)[65, 66]. Furthermore, the lower adsorption capacity of the Ni (II) ion may also be due to its lower covalent binding index (5.63) compared to the Pb (II) ion (11.1), so that Lead has a greater tendency to form covalent bonds with adsorbent ligands[67].
4. Conclusions
The characterization of biomaterials shows the presence of hydroxyl, carbonyl, and carboxyl groups, corresponding to cellulose and lignin, which are attributed to participating in the adsorption process. It was found that the best conditions for Ni removal were 1 mm, 0.0783 g of adsorbent and 45 ºC, while for Pb (II) they were 1 mm, 0.1166 g of adsorbent and 34 ºC. With these conditions, the best adsorption capacity for Pb and Ni with lemon peel were 304.5 mg/g and 72.03 mg/g, respectively. Adsorption kinetics was adjusted by the Pseudo-Second Order model and the Dubinin-Radushkevich model, a good fit was found for the Ni (II) and Pb (II) isotherm, suggesting that the process is mostly controlled by physisorption. From the thermodynamic study it was established that the adsorption process of Pb (II) is endothermic and that of Ni (II) is exothermic, and that the removal of both metals is irreversible. The binary study did not show competition between the ions for the active sites of the adsorbent, which may be related to the number of sites available and their selectivity by one of the cations.