Services on Demand
Journal
Article
Indicators
-
Cited by SciELO
-
Access statistics
Related links
-
Cited by Google
-
Similars in SciELO
-
Similars in Google
Share
Agronomía Colombiana
Print version ISSN 0120-9965
Agron. colomb. vol.33 no.2 Bogotá May/Aug. 2015
https://doi.org/10.15446/agron.colomb.v33n2.49846
Doi: 10.15446/agron.colomb.v33n2.49846
1 Department of Biology, Faculty of Sciences, Universidad Nacional de Colombia. Bogota (Colombia)
2 Oil Palm Biology and Breeding Research Program, Colombian Oil Palm Research Center (Cenipalma). Bogota (Colombia). hromero@cenipalma.org; hmromeroa@unal.edu.co
Received for publication: 26 March, 2015. Accepted for publication: 30 June, 2015.
Abstract
Water supply is the main limiting factor that affects oil palm (Elaeis guineensis Jacq.) yield. This study aimed to evaluate the gas exchange and photosynthetic capacity, determine the physiological effects and assess the tolerance potential of oil palm genotypes under water-deficit conditions. The two oil palm commercial genotypes IRHO1001 and IRHO7010 were exposed to soil water potentials of -0.042 MPa (field capacity or well-watered) or -1.5 MPa (drought-stressed). The leaf water potential and gas exchange parameters, including photosynthesis, stomatal conductance, transpiration and water use efficiency (WUE), as well as the photosynthesis reduction rate were monitored at 4 and 8 weeks after treatment. The IRHO7010 genotype showed fewer photosynthesis changes and a smaller photosynthetic reduction under the prolonged water deficit conditions of 23% at 4 weeks after the treatment as compared to 53% at 8 weeks after treatment, but the IRHO1001 genotype showed 46% and 74% reduction at the two sampling times. 'IRHO7010' had a higher stomatal conductance and transpiration potential than 'IRHO1001' during the water shortage. The WUE and leaf water potential were not different between the genotypes during dehydration. The data suggested that 'IRHO7010' had a higher photosynthetic capacity during the drought stress and was more drought-tolerant than 'IRHO1001'.
Key words: African oil palm, drought stress, photosynthesis capacity, susceptible, tolerant.
Resumen
El suministro de agua es la limitante principal del rendimiento de la palma de aceite (Elaeis guineensis Jacq.). El objeto de este trabajo fue estudiar el intercambio de gases y la capacidad fotosintética para determinar los efectos fisiológicos y evaluar el potencial de la tolerancia de dos genotipos de palma de aceite bajo condiciones de déficit de agua. Dos genotipos comerciales de palma aceitera, IRHO1001 e IRHO7010 fueron expuestos a -0.042 MPa (capacidad de campo o bien regado) y -1.5 MPa (estrés de sequía). El potencial hídrico de la hoja y los parámetros de intercambio de gases, incluyendo la fotosíntesis, conductancia estomática, transpiración y eficiencia del uso del agua (EUA) se revisaron a las 4 y 8 semanas después de iniciado el tratamiento considerando el porcentaje de reducción de fotosíntesis en cada tiempo. El genotipo IRHO7010 mostró menos cambios en la fotosíntesis y en la reducción fotosintética a las 4 semanas (23%) y 8 semanas (53%) de iniciado el tratamiento de déficit hídrico prolongado, en comparación al genotipo IRHO1001 que presentó una reducción de 46 y 74% para los muestreos de las 4 y 8 semanas, respectivamente. 'IRHO7010' tuvo mayor conductancia estomática y transpiración que 'IRHO1001' durante el déficit hídrico. La EUA y el potencial hídrico de la hoja no mostraron ninguna diferencia entre los dos genotipos durante la sequía. Los datos sugieren que 'IRHO7010' tiene mayor capacidad fotosintética durante el estrés por sequía y por tanto puede ser más tolerante a la sequía que 'IRHO1001'.
Palabras clave: palma de aceite africana, estrés de sequía, capacidad de fotosíntesis, susceptible, tolerante.
Introduction
During plant evolution, physiological regulators, such as stomatal and guard cell adjustment, chloroplast reactions, membrane depolarization and expression-to-function signaling, have been developed to save water and optimize water use for subsequent periods (Wasilewska et al., 2008; Sirichandra et al., 2009). These mechanisms enable plants to tolerate water deficits and, therefore, are of interest and importance for further studies. Research examining the soil-water-plant relationship during drought conditions improves our understanding of the physiological responses of plants to water deficits. These studies are important because the results can be used to genetically improve drought tolerance by discriminating among tolerant genotypes that behave better and more efficiently in terms of drought response (Zlatev and Lidon, 2012).
Photosynthesis is the process that plants employ to fix energy; so, a plant's yield and survival depend on their photosynthetic capacity (Lauteri et al., 2014; Ambavaram et al., 2014). Drought causes stomatal closure, available water reduction and impaired physiological reactions, reducing the photosynthetic rate (Mafakheri et al., 2010). Thus, the study of photosynthetic rates in stressed plants will help us to understand how plants tolerate water deficit conditions (Graça et al., 2010).
Stomatal closure is the first line of defense against dehydration (Hopper et al., 2014). Stomata are regulated based on the level of the water deficit and may partially close, allowing carbon fixation during drought conditions with improved water use efficiency (Benešová et al., 2012). These changes are caused by the low availability of water in the soil, resulting in plants retaining water and not losing it to the atmosphere. Water conservation prevents dryness, regulates the CO2 content with chemical and biochemical mechanisms and fixes more carbon with distinct pathways, such as stomatal adjustment (Gilbert et al., 2011; Nilsen et al., 2014). Thus, plants that possess better control of the stomatal function (closure/apertures) are more drought-tolerant.
Drought tolerance does not depend on a single physiological trait and is instead the relative contribution of several tolerance mechanisms functioning at different stages of plant development (Jaleel et al., 2009). There is a network of different mechanisms, such as stomatal conductance, photosynthetic potential, root system, osmotic adjustment and reserves of assimilates that plants adopt during drought conditions to survive and reduce the effects of water deficits (Nieto-Garibay et al., 2009; Farooq et al., 2009; Rivera et al., 2012).
Water deficit stress reduces plant yield and production and is the main limiting factor for oil palm productivity (Kallarackal et al., 2004; Rivera et al., 2012). Oil palms need 4-5 mm of water daily and demand 1,800-2,000 mm of annual precipitation for optimum production. There is a 10% decrease in oil palm production for every 100 mm of water reduction due to rainfall shortages (Carr, 2011). Thus, long-term drought periods drastically reduce oil palm productivity.
Oil palm cultivated areas worldwide are suffering from water availability problems. Colombia is the fourth oil palm producing country worldwide, after Indonesia, Malaysia and Thailand, and the leading producer in Latin America (FAO, 2013). Three of the four oil palm-growing zones, including the Northern, Eastern and Central regions, face prolonged drought periods of 4 to 8 months (Romero et al., 2007; Rivera et al., 2012).
There is a direct reduction of up to 20% in the yield of fresh fruit bunches (Caliman and Southworth, 1998) and oil (Cornaire et al., 1994) due to water deficits. Drought periods may affect the male and female inflorescence ratio, decreasing the bunch number and, accordingly, production (Corley and Tinker, 2015). The water problem for oil palms is the result of climate changes that reduce precipitation and increase drought seasons. Additionally, it is difficult to develop and utilize irrigation systems for large areas to avoid water deficit problems or to reduce drought period impacts on oil palms because of economic, technical and agricultural limitations (Rivera et al., 2012).
Understanding oil palm behavior and responses to water deprivation is very important and can explain oil palm physiological patterns and responses upon exposure to drought conditions. Therefore, physiological and genetic studies that examine how oil palm genotypes respond to droughts constitute one of the better options for identifying high-performing plants that tolerate water shortages (Silva et al., 2013).
This comparative study examined the gas exchange responses and photosynthetic capacity of two commercial oil palm genotypes cultivated under water deficit conditions during their initial growth phase. The two oil palm genotypes, known as IRHO7010 and IRHO1001, are cultivated in Colombia due to their potential production ability and disease tolerance (Louise et al., 2007). We determined which genotype performed better physiologically under a prolonged water shortage; that is, which was more drought-tolerant. These findings can be used in subsequent studies on oil palm drought stress, such as genetic, transcriptomic and breeding programs to evaluate the molecular responses of oil palms to water deficits and to screen for tolerant genotypes.
Materials and method
Study location and plant materials
The study was conducted in a mesh house located at the "El Palmar de La Vizcaína" Experimental Field, Barrancabermeja-Santander, Colombia. The tropical agroecological attributes included an average temperature of 34°C, 70.5% relative humidity and precipitation of 3,800 mm yr-1.
Two oil palm genotypes that were bred by the CIRAD institute (France) and that are cultivated in Colombia were used in the present study: IRHO 7010 ((DA 115 D × DA 3 D) × LM 2 T × LM 10 T) and IRHO1001 (DA 115 D AF x LM 2 T AF) (Louise et al., 2007).
The pre-germinated palm seeds were planted in plastic containers consisting of a tube with a diameter of 25 cm and a length of 50 cm filled with a soil composed of 18.6% fine sand, 40.7% clay and 40.7% silt. The density of the soil was 1.2 g cm-3. Saturating irrigation was performed for 3 d to compact the soil and homogenize the soil structure. The irrigation system was then installed, and the soil was kept at FC (field capacity) for further treatments. The FC and wilting point (WP) or permanent wilting point (PWP) were calculated according to Saxton and Rawls (2006); the FC and WP were approximately 38% (%V) and 22%, respectively, considering the silty clay loam texture of the studied soil. These values were used to determine the non-stress and severe drought condition for the study.
The soil water retention was determined based on the equation introduced by Da Silva et al. (1994) (Eq. 1). The water content at FC was calculated by saturating the soil using either volumetric or weight-based methods and then the PWP or WP was calculated.

where, PWP is the permanent wilting point, DW is the dry weight of the soil and FC is the field capacity.
Drought treatment
The irrigation system for each of the containers was an 8 L h-1 capacity dropper connected to a 3-mm drip hose. Each container was irrigated with four droppers installed in four corners of the container to ensure a maximum irrigation depth of 10 cm and to maximize and homogenize the irrigation coverage.
The pre-germinated seeds were maintained in a pre-nursery bag for 30 d until two lanceolate leaves appeared, according to phenology growth stage 102 (Hormaza et al., 2012). The plants were transplanted to the containers, where they were maintained at FC for 30 d to adapt to the new condition. To maintain a constant soil tension and water potential, the irrigation program applied in each of the planned water potentials was calculated considering the effective root depth and physical characteristics of the soil, including texture, bulk density and moisture retention curve.
The soil moisture was monitored daily using an SM200 (Delta T-Devices, Cambridge, UK) sensor coupled to a manual Data Logger HH2 (Delta T-Devices, Cambridge, UK). The FC was measured on a dry basis and its equivalence was set to a water potential of -0.042 MPa. Following the adaptation period, the plants were subjected to two soil water potentials: -0.042 MPa (as the field capacity or control, well-watered: WW) and -1.50 MPa (as severe water deficit, drought treatment: DT) by withholding water. The evaluated time points were 4 and 8 weeks after treatment (WAT). A completely randomized 2 x 2 factorial design array experiment was used with three replications and 4 plants per replication.
Leaf water potential
The leaf water potential was determined using the Plant Water Status Console device, Model 3005 (Soilmoisture Equipment, Santa Barbara, CA). The measurements were taken in the third leaf between 9:00 and 11:30 AM.
Gas exchange measurements
The measurements of photosynthesis (Pn) (mmol CO2 m-2 s-1), stomatal conductance (gs) (mol H2O m-2 s-1) and transpiration (E) (mmol H2O m-2 s-1) were recorded using an LI-6400XT open-path Portable Photosynthesis System (LI-COR, Lincoln, NE). The following parameters were fixed during the measuring points: CO2 concentration in the chamber: 400 mg L-1; Flux: 170 mol s-1, and PAR: 1,000 mmol m2 s-1. The measurements were taken on the third leaf of the palms in the morning between 9:00 and 11:30 AM. The water use efficiency (WUE) (mol CO2/mmol H2O) (Pn/E) was calculated. The reduction in the photosynthetic rate was determined at the desired time points in order to differentiate the two genotypes.
Statistical analyses
The experimental data were subjected to analyses of variance and mean comparison using the Student's t-test with a 5% probability, employing SAS® statistical software, Version 9.1 (SAS Institute, Cary, NC). Multibase2015 (www.numericaldynamics.com) was used to discriminate the plant genotypes using the PCA (principal component analysis) option.
Results
Soil water retention
The water potentials for the FC and WP of the soil were calculated as -0.042 and -2.400 MPa, respectively. These values were equivalent to an FC (%Volumetric) of approximately 39% and a WP of approximately 23% (Tab. 1). After calculating the WP for the soil, -1.5 MPa was considered severe drought, which was the preferred point used to determine the physiological parameters. Thus, the drought treatment corresponded to a soil water potential greater than the WP; so, the oil palm tolerance level to drought was assessed before reaching the WP. Figure 1 shows the soil water-retention curve obtained for the studied soil in accordance with Eq. 1.
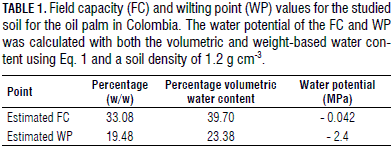
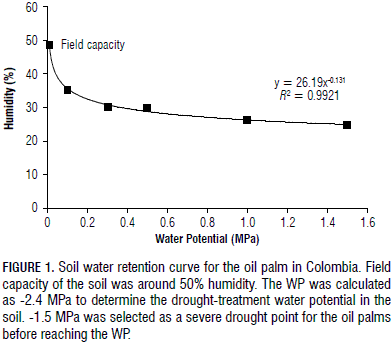
Leaf water potential
There was a significant difference for both genotypes at the studied times in terms of leaf water potential between the well-watered and drought-treated plants. The DT plants reached more negative potentials in the prolonged drought condition (Fig. 2). However, they decreased after 4 weeks of treatment in both genotypes and were constant during the stress between 4 to 8 weeks, indicating that, during this time, the water potential in the soil was maintained as a constant; the leaf water potential also remained constant.
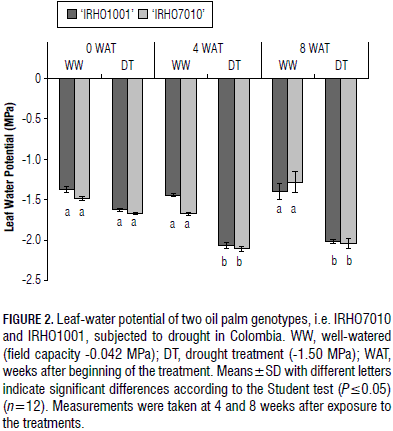
There were no differences in the leaf water potentials between the sampling times, i.e. 4 and 8 WAT, in the genotypes (Fig. 2). The leaf water potential for the WW plants of both genotypes was approximately constant and did not show any significant difference during the sampling times, 0, 4 and 8 WAT (Fig. 2).
Gas exchange parameters
The gas exchange parameters differed between the two genotypes in both the WW and DT plants at both time points, i.e. 4 and 8 WAT. At the beginning of the study, all values were similar and there were no significant differences for the two genotypes and two conditions. The WW treatment did not cause any significant differences during the study (in 0, 4 and 8 WAT) for any of the studied parameters in either genotype.
The two genotypes experienced a reduction in photosynthesis during the prolonged water shortage, but there were different gradients and rates (Fig. 3). The 'IRHO1001' plants exhibited a faster decline in the photosynthetic rate, from 13.34 to 6.72 mmol CO2 m-2 s-1 (46% reduction), after 4 weeks of drought treatment. Photosynthesis continued to decline during the additional 4 weeks of drought and reached 3.03 mmol CO2 m-2 s-1 (74% reduction). The 'IRHO7010' plants lost photosynthetic activity slowly. Photosynthesis changed from 13.03 at zero point to 8.25 mmol CO2 m-2 s-1 (23% reduction) and 5.73 mmol CO2 m-2 s-1 (53% reduction) at 4 and 8 weeks of water shortage, respectively. The WW plants did not have a significant change in their photosynthesis during the period of measurements.
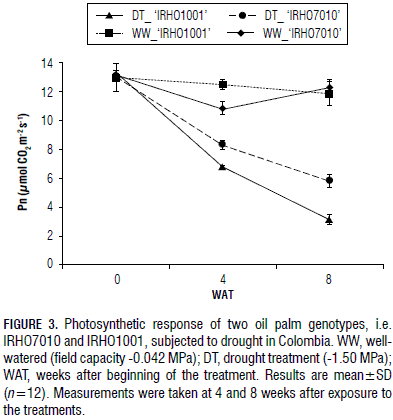
The drought treatment caused a decreased in stomatal conductance(gs) in the 'IRHO1001' plants, from 0.35 mol H2O m-2 s-1 to 0.15 mol H2O m-2 s-1, after 4 weeks of treatment. In the same treatment period, the 'IRHO7010' gs dropped from 0.36 mol H2O m-2 s-1 to 0.20 mol H2O m-2 s-1 (Fig. 4). At 8 WAT, the gs was further reduced in both genotypes, reaching 0.05 mol H2O m-2 s-1 in 'IRHO1001' and 0.07 mol H2O m-2 s-1 in 'IRHO7010'. A significant difference was seen for gs between the two genotypes in the drought condition. There was also a difference between the genotypes at different times. The gs for the two genotypes behaved differently between the WW and DT. Thus, the IRHO7010 genotype more efficiently adjusted its stomata and was able to maintain the stomata opened, resulting in continued gas exchange and CO2 assimilation.
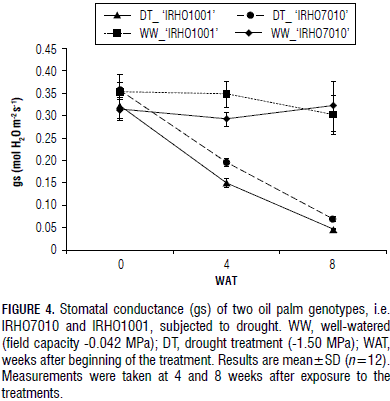
The transpiration (E) was also affected by the drought treatment. In 'IRHO1001', it fell more rapidly than in 'IRHO7010' at 4 and 8 WAT. The 'IRHO1001' DT plants showed 5.21, 2.83 and 1.38 mmol H2O m-2 s-1 for the transpiration values at 0, 4 and 8 WAT, respectively. The values for the WW 'IRHO1001' plants at 0, 4 and 8 WAT were 5.32, 4.14 and 4.59 mmol H2O m-2 s-1, respectively (Fig. 5). For the 'IRHO7010' plants, E was 5.44, 3.20 and 1.87 mmol H2O m-2 s-1 for the DT plants and 5.12, 3.90 and 4.19 mmol H2O m-2 s-1 for the WW plants at 0, 4 and 8 WAT, respectively. A significant reduction was seen at each time point when comparing the WW and DT samples of both genotypes and also when comparing the two genotypes with each other in the drought condition of the same time, i.e. the DT plants in 4 and 8 WAT. No difference was seen between the genotypes for field capacity (WW).
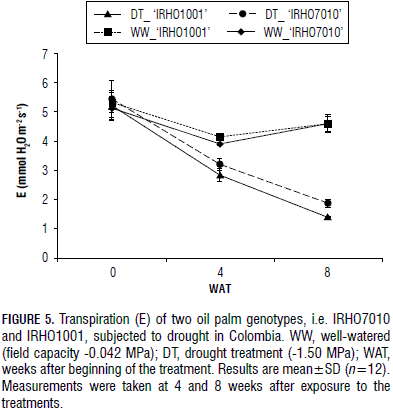
The 'IRHO1001' plants showed a decrease in water use efficiency (WUE) of approximately 20%, and 'IRHO7010' exhibited a 6% reduction after 4 WAT. At 8 WAT, 'IRHO7010' showed an increase of 12% and a 14% decrease was observed for 'IRHO1001' (Fig. 6). These data indicate that 'IRHO1001' experienced a reduced photosynthetic rate while maintaining high transpiration, which caused the WUE to decrease. Conversely, 'IRHO7010' controlled the transpiration rate more efficiently and maintained a high net photosynthesis rate and WUE.
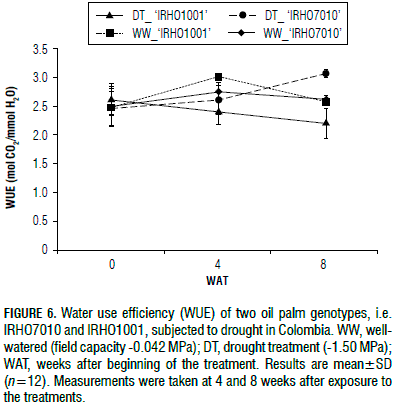
Grouping genotypes
Photosynthesis (Pn) and its reduction rate percentage, as well as E and gs, were the parameters that showed significant differences between the WW and DT samples for each genotype as depicted in Fig. 7. The two genotypes were found to be very different based on the DT samples and they behaved differently in terms of drought responses, as they were separately grouped by their physiological parameters (Fig. 7). The WW samples for both genotypes ranged very near each other, indicating that the WW samples behaved similarly in both genotypes. The results indicate that the WW plants were similar for all of the studied physiological parameters (Fig. 7).
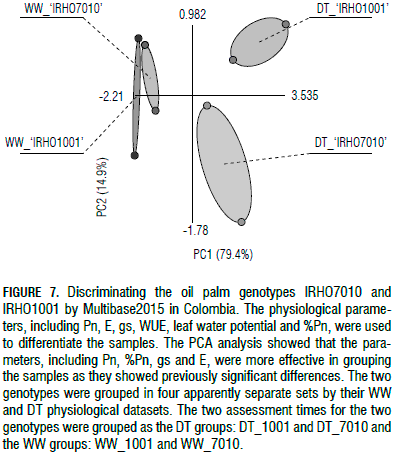
Discussion
A water potential less than -1.5 MPa has been considered as severe drought condition for many plants because it can affect physiological variables harshly, decreasing photosynthesis and stomatal conductance, changing some metabolites and regulating gene expression to mitigate water deficit effects (Vásquez-Robinet et al., 2010; Liu et al., 2011; Kakumanu et al., 2012). In the current study, the soil water potential of -1.5 MPa was considered a severe drought condition as previously reported for oil palms (Rivera et al., 2012) and showed the analogous response patterns for physiological parameters, confirming its severity for oil palms as well.
Photosynthesis was used as the main parameter to evaluate tolerance levels to water deficits and to screen drought-tolerant genotypes because this parameter is directly related to plant water shortage responses (Pinheiro and Chaves, 2011; Zlatev and Lidon, 2012; Ashraf and Harris, 2013; Zhou et al., 2013). The results showed that it can indeed be used to discriminate oil palm genotypes with different drought tolerance.
The studied parameters for the WW oil palm plants of both genotypes were stable during the study, confirming that the WW plants were in similar optimum conditions and maintained acceptable water contents. Significant differences were observed for the photosynthetic parameters, which suggested that 'IRHO7010' decreased its photosynthesis activity by around 50% during the prolonged water deficit period and could perform photosynthesis better than 'IRHO1001', which reduced its photosynthesis efficiency by more than 74% (Fig. 3). During the prolonged drought, 'IRHO7010' significantly exhibited a smaller decrease in the photosynthetic rate than 'IRHO1001' for the same drought treatment and time. These findings indicate that the IRHO1001 genotype is less tolerant to water shortages and reacted to water availability by substantially decreasing its photosynthesis rate, while the IRHO7010 genotype, as a tolerant genotype, managed a better photosynthesis performance under the water deficit conditions.
Water deficit conditions influence plants in terms of the physiological processes involved in growth, development and production. Droughts can drastically reduce plant yield by impacting the principal gas exchange mechanisms and photosynthesis (Lawlor and Tezara, 2009; Centritto et al., 2009). Thus, tolerant plants can manage their physiological parameters, especially photosynthesis, better (Hura et al., 2007; Chaves et al., 2009; Pinheiro and Chaves, 2011; Ji et al., 2012; Barnaby et al., 2013), as was observed in the present study.
The results indicate that 'IRHO7010' could adjust stomatal conductance better than 'IRHO1001' (Fig. 4). Thus, the IRHO7010 genotype, with a higher stomatal control was more drought-tolerant. This kind of behavior has been reported previously in other plants, in which tolerant plants maintained photosynthesis and more gas exchanges to have enough CO2 for photosynthetic reactions than non-tolerant plants (Heschel and Riginos, 2005; Gilbert et al., 2011; Zhou et al., 2013). Drought-tolerant plants can employ stomata in favor of making CO2 more available, which is used to perform photosynthesis under drought conditions as seen in 'IRHO7010', but not in 'IRHO1001'.
The changes in transpiration followed the same patterns as photosynthesis and stomatal conductance in the two genotypes. The 'IRHO7010' plants exhibited a higher transpiration rate than the 'IRHO1001' plants at both assessment time points (Fig. 5). However, the difference between the values of E and gs for the WW and DT plants showed less change in 'IRHO7010', indicating that it could control gas exchange more effectively than 'IRHO1001'. These results suggest that 'IRHO7010' could manage the drought condition better than 'IRHO1001' by adjusting its gas exchange and stomatal control.
There was a positive relationship between the stomatal conductance and transpiration in response to the low water availability. This behavior has been evaluated and reported previously in different plant genotypes under water shortage conditions (Cha-um et al., 2010; Rahbarian et al., 2011; Silva et al., 2013). The parallel changes in the studied parameters improved the efficiency of the physiological responses in the IRHO7010 genotype, found as the tolerant genotype. These data are concordant with previously reported data for sugarcane (Silva et al., 2013), soybean (Fenta et al., 2012) and maize (Benešová et al., 2012), where more tolerant plants showed less inhibition of the transpiration and stomatal conductance and a smaller decrease of photosynthetic performance during droughts.
As shown in Fig. 6, the WUE did not show any significant differences between the two studied oil palm genotypes. These non-significant changes could be caused by the relationship between photosynthesis and stomatal and the non-stomatal control of CO2 diffusion. The WUE of the IRHO7010 genotype after 4 WAT started to increase; however, it was not significantly different. The data suggest that the 'IRHO7010' plants could employ other internal mechanisms to maintain photosynthesis, transpiration and stomatal conductance efficiency. These mechanisms could include molecular agents that mitigate the effects of drought on these variables, such as transcription factors involved in stomatal activity and regulation of photosynthesis, photosystem II repair, rubisco activity and scavenging of reactive oxygen species (ROS) (Saibo et al., 2009).
The WUE can reveal how a plant is able to fix carbon during drought conditions based on the water used (Bacon, 2004), but it varies because of different factors, such as leaf spatial orientation, seasonal effects, nocturnal and diurnal molecular responses, and single leaf vs. whole plant study scale, etc. (Medrano et al., 2015). Because of its variation, the WUE cannot be used solely to determine drought tolerance as other variables can, i.e. photosynthesis and transpiration, but it may be used to complete the results of photosynthesis and transpiration studies during water shortages.
As shown in Fig. 7, the samples were successfully categorized by Multibase2015 using a PCA analysis according to their physiological responses into four different groups, including two genotypes by two water treatments separately. The WW plants were completely different from the DT plants in both genotypes and the DT plants were totally distinct between both genotypes. These findings suggest that the two genotypes behaved differently in terms of the studied physiological parameters and were successfully classified based on their drought-tolerance levels, i.e. 'IRHO7010' as tolerant and 'IRHO1001' as susceptible.
Conclusion
This study examined two oil palm genotypes for their drought tolerance by evaluating physiological variables, including photosynthesis, stomatal conductance, transpiration, water use efficiency and leaf water potential. The photosynthetic rate was used to differentiate the genotypes. This characteristic was ascribed to the ability of 'IRHO7010' to control water in the leaves. Higher stomatal conductance and transpiration were observed during the prolonged water shortage in 'IRHO7010'. The photosynthesis reduction rate showed a slower diminishing rate in the 'IRHO7010' plants than those of 'IRHO1001'. Thus, our results suggest that 'IRHO7010' was able to mitigate the prolonged drought conditions more effectively than 'IRHO1001' during the initial phase of development. 'IRHO7010' is considered as more drought-tolerant than 'IRHO1001', according to the obtained results.
These results can be used in downstream studies on these oil palm genotypes in omics studies, such as genomics and transcriptomics to find likely genes, pathways, processes and mechanisms which are involved in oil palm drought tolerance and in terms of oil palm plant breeding programs based on molecular responses.
The strategy of studying changes of desired physiological parameters between WW and DT plants (i.e. reduction percent of photosynthesis, transpiration and stomatal conductance) as a whole package seems more efficient for deducing tolerance levels in different genotypes, rather than studying each parameter individually and separately. 'IRHO7010', as the more tolerant genotype, can be recommended for cultivation in Colombian oil palm plantations, where water deficits are a regional problem. Of course, the production and yield potential of both genotypes should be taken into account in further studies to more effectively support this suggestion.
Acknowledgments
Research at Cenipalma is funded by the Colombian Oil Palm Promotion Fund (FFP), administered by Fedepalma (FederaciónNacional de Cultivadores de Palma de Aceite). The authors would like to thank Mr. Cristihian Jarri Bayona Rodriguez for his scientific support, Mr. Wilson Diaz Castillo for his field assistance at Cenipalma and Mrs. Eloina Mesa Fuquen for her assistance with the statistical analyses.
Literature cited
Ambavaram, M.M.R., S. Basu, A. Krishnan, V. Ramegowda, U. Batlang, L. Rahman, N. Baisakh, and A. Pereira. 2014. Coordinated regulation of photosynthesis in rice increases yield and tolerance to environmental stress. Nat. Commun. 5, 1-14. Doi: 10.1038/ncomms6302 [ Links ]
Ashraf, M. and P.J.C. Harris. 2013. Photosynthesis under stressful environments: an overview. Photosynthetica 51, 163-190. Doi: 10.1007/s11099-013-0021-6 [ Links ]
Bacon, M.A. 2004. Water use efficiency in plant biology. pp. 1-26. In: Bacon, M.A. (ed.). Water use efficiency in plant biology. Blackwell Publishing, Oxford, Uk. [ Links ]
Barnaby, J.Y., M. Kim, G. Bauchan, J. Bunce, V. Reddy, and R.C. Sicher. 2013. Drought responses of foliar metabolites in three maize hybrids differing in water stress tolerance. PLoS One 8(10), e77145. Doi: 10.1371/journal.pone.0077145 [ Links ]
Benešová, M., D. Holá, L. Fischer, P.L. Jedelsky, F. Hnilička, N. Wilhelmová, O. Rothová, M. Kočová, D. Procházková, J. Honnerová, L. Fridrichová, and H. Hniličková. 2012. The physiology and proteomics of drought tolerance in maize: early stomatal closure as a cause of lower tolerance to short-term dehydration? PLoS One 7(6), e38017. Doi: 10.1371/journal.pone.0038017 [ Links ]
Caliman, J.-P. and A. Southworth. 1998. Effect of drought and haze on the performance of oil palm. In: International Oil Palm Conference. IOPRI, Bali, Indonesia. [ Links ]
Carr, M.K.V. 2011. The water relations and irrigation requirements of oil palm (Elaeis guineensis): a review. Exp. Agric. 47, 629-652. Doi: 10.1017/S0014479711000494 [ Links ]
Centritto, M., M. Lauteri, M.C. Monteverdi, and R. Serraj. 2009. Leaf gas exchange, carbon isotope discrimination, and grain yield in contrasting rice genotypes subjected to water deficits during the reproductive stage. J. Exp. Bot. 60, 2325-39. Doi: 10.1093/jxb/erp123 [ Links ]
Cha-um, S., T. Takabe, and C. Kirdmanee. 2010. Osmotic potential, photosynthetic abilities and growth characters of oil palm (Elaeis guineensis Jacq.) seedlings in responses to polyethylene glycol-induced water deficit. Afr. J. Biotechnol. 9, 6509-6516. [ Links ]
Chaves, M.M., J. Flexas, and C. Pinheiro. 2009. Photosynthesis under drought and salt stress: regulation mechanisms from whole plant to cell. Ann. Bot. 103, 551-60. Doi: 10.1093/aob/mcn125 [ Links ]
Corley, R.H.V. and P.B.H. Tinker. 2015. The oil palm. 5th ed. Wiley-Blackwell, Oxford, UK. [ Links ]
Cornaire, B., C. Daniel, Z. Fodil, and E. Lamade. 1994. Comportamiento de la palma de aceite bajo estrés hídrico. Palmas 15, 61-70. [ Links ]
Da Silva, A.P., B.D. Kay, and E. Perfect. 1994. Characterization of the least limiting water range of soils. Soil Sci. Soc. Am. J. 58, 1775-1781. Doi: 10.2136/sssaj1994.03615995005800060028x [ Links ]
FAO. 2013. FAOSTAT. In: http://faostat.fao.org/default.aspx; consulted: May, 2015. [ Links ]
Farooq, M., A. Wahid, N. Kobayashi, D. Fujita, and S.M.A. Basra. 2009. Plant drought stress: effects, mechanisms and management. Agron. Sustain. Dev. 29, 185-212. Doi: 10.1051/agro:2008021 [ Links ]
Fenta, B.A., S.P. Driscoll, K.J. Kunert, and C.H. Foyer. 2012. Characterization of drought-tolerance traits in nodulated soya beans: the importance of maintaining photosynthesis and shoot biomass under drought-induced limitations on nitrogen metabolism. J. Agron. Crop Sci. 198, 92-103. Doi: 10.1111/j.1439-037X.2011.00491.x [ Links ]
Gilbert, M.E., M.A. Zwieniecki, and N.M. Holbrook. 2011. Independent variation in photosynthetic capacity and stomatal conductance leads to differences in intrinsic water use efficiency in 11 soybean genotypes before and during mild drought. J. Exp. Bot. 62, 2875-2887. Doi: 10.1093/jxb/erq461 [ Links ]
Graça, J.P. da, F.A. Rodrigues, J.R.B. Farias, M.C.N. de Oliveira, C.B. Hoffmann-Campo, and S.M. Zingaretti. 2010. Physiological parameters in sugarcane cultivars submitted to water deficit. Braz. J. Plant Physiol. 22, 189-197. Doi: 10.1590/S1677-04202010000300006 [ Links ]
Heschel, M.S. and C. Riginos. 2005. Mechanisms of selection for drought stress tolerance and avoidance in Impatiens capensis (Balsaminaceae). Am. J. Bot. 92, 37-44. Doi: 10.3732/ajb.92.1.37 [ Links ]
Hopper, D.W., R. Ghan, and G.R. Cramer. 2014. A rapid dehydration leaf assay reveals stomatal response differences in grapevine genotypes. Hortic. Res. 1, 2. Doi: 10.1038/hortres.2014.2 [ Links ]
Hormaza, P., E.M. Fuquen, and H.M. Romero. 2012. Phenology of the oil palm interspecific hybrid Elaeis oleifera x Elaeis guineensis. Sci. Agric. 69, 275-280. Doi: 10.1590/S0103-90162012000400007 [ Links ]
Hura, T., S. Grzesiak, K. Hura, E. Thiemt, K. Tokarz, and M. Wedzony. 2007. Physiological and biochemical tools useful in drought-tolerance detection in genotypes of winter triticale: accumulation of ferulic acid correlates with drought tolerance. Ann. Bot. 100, 767-775. Doi: 10.1093/aob/mcm162 [ Links ]
Jaleel, C.A., P. Manivannan, A. Wahid, M. Farooq, R. Somasundaram, R. Panneerselvam, and F.L. Article. 2009. Drought stress in plants: a review on morphological characteristics and pigments composition. Int. J. Agric. Biol. 11, 100-105. [ Links ]
Ji, K., Y. Wang, W. Sun, Q. Lou, H. Mei, S. Shen, and H. Chen. 2012. Drought-responsive mechanisms in rice genotypes with contrasting drought tolerance during reproductive stage. J. Plant Physiol. 169, 336-344. Doi: 10.1016/j.jplph.2011.10.010 [ Links ]
Kakumanu, A., M.M.R. Ambavaram, C. Klumas, A. Krishnan, U. Batlang, E. Myers, R. Grene, and A. Pereira. 2012. Effects of drought on gene expression in maize reproductive and leaf meristem tissue revealed by RNA-Seq. Plant Physiol. 160, 846-67. Doi: 10.1104/pp.112.200444 [ Links ]
Kallarackal, J., P. Jeyakumar, and S.J. George. 2004. Water use of irrigated oil palm at three different arid locations in Peninsular India. J. Oil Palm Res. 16, 45-53. [ Links ]
Lauteri, M., M. Haworth, R. Serraj, M.C. Monteverdi, and M. Centritto. 2014. Photosynthetic diffusional constraints affect yield in drought stressed rice cultivars during flowering. PLoS One 9(9), e109054. Doi: 10.1371/journal.pone.0109054; 10.1371/journal.pone.0117631 [ Links ]
Lawlor, D.W. and W. Tezara. 2009. Causes of decreased photosynthetic rate and metabolic capacity in water-deficient leaf cells: a critical evaluation of mechanisms and integration of processes. Ann. Bot. 103, 561-579. Doi: 10.1093/aob/mcn244 [ Links ]
Liu, C., Y. Liu, K. Guo, D. Fan, G. Li, Y. Zheng, L. Yu, and R. Yang. 2011. Effect of drought on pigments, osmotic adjustment and antioxidant enzymes in six woody plant species in karst habitats of southwestern China. Environ. Exp. Bot. 71, 174-183. Doi: 10.1016/j.envexpbot.2010.11.012 [ Links ]
Louise, C., P. Amblard, H. de Franqueville, D. Benavides, and C. Gallardo. 2007. Investigaciones dirigidas por el CIRAD sobre las enfermedades del complejo pudrición del cogollo de la palma aceitera en Latinoamérica. Palmas 28(Special Issue), 345-362. [ Links ]
Mafakheri, A., A. Siosemardeh, B. Bahramnejad, P.C. Struik, and Y. Sohrabi. 2010. Effect of drought stress on yield, proline and chlorophyll contents in three chickpea cultivars. Aust. J. Crop Sci. 4, 580-585. [ Links ]
Medrano, H., M. Tomás, S. Martorell, J. Flexas, E. Hernández, J. Rosselló, A. Pou, J.-M. Escalona, and J. Bota. 2015. From leaf to whole-plant water use efficiency (WUE) in complex canopies: limitations of leaf WUE as a selection target. Crop J. 3, 220-228. Doi: 10.1016/j.cj.2015.04.002 [ Links ]
Nieto-Garibay, A., E. Troyo-Diéguez, J.L. García-Hernández, B. Murillo-Amador, F.H. Ruiz-Espinoza, and E. Pimienta-Barrios. 2009. Efecto del estrés hídrico edáfico en emergencia y desarrollo de plántula en las especies de chile (Capsicum frutescens L. y Capsicum annuum L.). Trop. Subtrop. Agroecosyst. 10, 405-413. [ Links ]
Nilsen, E.T., J. Freeman, R. Grene, and J. Tokuhisa. 2014. A rootstock provides water conservation for a grafted commercial tomato (Solanum lycopersicum L.) line in response to mild-drought conditions: a focus on vegetative growth and photosynthetic parameters. PLoS One 9(12), e115380. Doi: 10.1371/journal.pone.0115380 [ Links ]
Pinheiro, C. and M.M. Chaves. 2011. Photosynthesis and drought: can we make metabolic connections from available data? J. Exp. Bot. 62, 869-882. Doi: 10.1093/jxb/erq340 [ Links ]
Rahbarian, R., R. Khavari-Nejad, A. Ganjeali, A. Bagheri, and F. Najafi. 2011. Drought stress effects on photosynthesis, chlorophyll fluorescence and water relations in tolerant and susceptible chickpea (Cicer arietinum L.) genotypes. Acta Biol. Cracov. Ser. Bot. 53, 47-56. Doi: 10.2478/v10182-011-0007-2 [ Links ]
Rivera M., Y.D., L. Moreno Ch., C.J. Bayona, and H.M. Romero. 2012. Physiological response of oil palm interspecific hybrids (Elaeis oleifera HBK Cortes versus Elaeis guineensis Jacq.) to water deficit. Braz. J. Plant Physiol. 24, 273-280. Doi: 10.1590/S1677-04202012000400006 [ Links ]
Romero, H.M., I.M. Ayala D., and R. Ruíz R. 2007. Ecofisiología de la palma de aceite. Palmas 28(Special Issue), 176-184. [ Links ]
Saibo, N.J.M., T. Lourenço, and M.M. Oliveira. 2009. Transcription factors and regulation of photosynthetic and related metabolism under environmental stresses. Ann. Bot. 103, 609-623. Doi: 10.1093/aob/mcn227 [ Links ]
Saxton, K.E. and W.J. Rawls. 2006. Soil Water characteristic estimates by texture and organic matter for hydrologic solutions. Soil Sci. Soc. Am. J. 70, 1569-1578. Doi: 10.2136/sssaj2005.0117 [ Links ]
Silva, M. de A., J.L. Jifon, C.M. dos Santos, C.J. Jadoski, and J.A.G. da Silva. 2013. Photosynthetic capacity and water use efficiency in sugarcane genotypes subject to water deficit during early growth phase. Braz. Arch. Biol. Technol. 56, 735-748. Doi: 10.1590/S1516-89132013000500004 [ Links ]
Sirichandra, C., A. Wasilewska, F. Vlad, C. Valon, and J. Leung. 2009. The guard cell as a single-cell model towards understanding drought tolerance and abscisic acid action. J. Exp. Bot. 60, 1439-1463. Doi: 10.1093/jxb/ern340 [ Links ]
Vásquez-Robinet, C., J.I. Watkinson, A.A. Sioson, N. Ramakrishnan, L.S. Heath, and R. Grene. 2010. Differential expression of heat shock protein genes in preconditioning for photosynthetic acclimation in water-stressed loblolly pine. Plant Physiol. Biochem. 48, 256-264. Doi: 10.1016/j.plaphy.2009.12.005 [ Links ]
Wasilewska, A., F. Vlad, C. Sirichandra, Y. Redko, F. Jammes, C. Valon, N. Frei dit Frey, and J. Leung. 2008. An update on abscisic acid signaling in plants and more... Mol. Plant 1, 198-217. Doi: 10.1093/mp/ssm022 [ Links ]
Zhou, S., R.A. Duursma, B.E. Medlyn, J.W.G. Kelly, and I.C. Prentice. 2013. How should we model plant responses to drought? An analysis of stomatal and non-stomatal responses to water stress. Agric. For. Meteorol. 182-183, 204-214. Doi: 10.1016/j.agrformet.2013.05.009 [ Links ]
Zlatev, Z. and F.C. Lidon. 2012. An overview on drought induced changes in plant growth, water relations and photosynthesis. Emir. J. Food Agric. 24, 57-72. [ Links ]