Introduction
SARS-CoV-2, the causative agent of COVID-19, is a virus with a high spreading capacity (1). By May 2022, there were more than 530 million confirmed COVID-19 cases and more than 6.2 million deaths related to this disease were reported worldwide (2). In Colombia, the first case was confirmed in March 2020. More than 6 million people have been infected, and around 140,000 people have died (3).
There is a broad spectrum of clinical manifestations in patients who acquire this infection. Patients produce high levels of proinflammatory cytokines (4) and, those with severe disease and admitted to the intensive care unit, have high plasma cytokines levels, including IL-7, IL-10, G-CSF, IP-10, MCP-1, MIP-1α, and TNF-α, compared to patients who develop mild or moderate disease (5). This cytokine storm is associated with increased vascular permeability, fluid accumulation, and the presence of blood cells in the alveoli, causing dyspnea and both respiratory and multiorgan failure, characteristic of patients with severe manifestations of COVID-19 (4).
Vaccines continue to be the most intensely researched strategy worldwide. However, approved vaccines face production, delivery and supply chain challenges, especially in developing countries. Recently, a fourth dose of the SARS-CoV-2 vaccine has been suggested to boost protection against SARS-CoV-2 new variants, putting additional pressure on vaccination programs worldwide (6). In addition, new variants, such as the delta and omicron variants, have potential of immune evasion and lower sensitivity to antibody neutralization, highlighting the importance of continuing the study of antiviral drugs (7-9). Consequently, antiviral agents are still necessary to reduce complications and mortality in those who acquire the infection (10).
Some antivirals and immunomodulatory drugs, such as hydroxychloroquine, were assayed as alternatives for severe cases. However, a low improvement of the clinical status was observed (11). The development of novel antiviral agents that can mitigate the pandemic represents a key research field. Metal nanoparticles represent an exciting approach since their antiviral action has been demonstrated in recent decades. There is evidence of the antiviral effect of metal nanoparticles against different viruses that include influenza, HIV, and hepatitis B (12).
Silver nanoparticles (AgNPs) have drawn the scientific community’s attention among different nanomaterials due to their proven antiviral action. Antiviral mechanisms are still unclear, but some studies suggest that these compounds can block viral binding and reduce viral infectivity. AgNPs interact with HIV gp120 and block its binding to CD4 molecules (13,14). In cultures of human hepatoma cells, these compounds inhibit the production of the hepatitis B virus (15). Interestingly, in MDCK cells, the infectivity of the influenza virus was reduced in cells treated with AgNPs, probably by a mechanism related to blocking the fusion of the virus with the host cell (16,17).
More recently, Almanza-Reyes et al. observed that AgNPs tested in vitro presented an inhibitory effect on SARS-CoV-2 infection in cultured cells. Additionally, they evaluated the effects of mouthwash and nose rinse with AgNPs, in the prevention of SARS-CoV-2 contagion in healthcare personnel, observing that AgNPs prevented SARS-CoV-2 infection when compared with the control group which performed conventional mouthwash and nose rinse (18).
In this study we synthesized, characterized, and evaluated the cytotoxic effect and the antiviral activity of AgNPs against SARS-CoV-2 in vitro.
Materials and methods
Cell lines and virus
Vero E6 cell line from Cercopithecus aethiops kidney was donated by Instituto Nacional de Salud (INS) (Bogotá, Colombia). The cells were maintained in Dulbecco’s modified Eagle medium (DMEM) supplemented with 10% FBS and 1% penicillin-streptomycin. Cultures were maintained at 37°C, with 5% CO2, whit three passages per week. The infections were done with a SARS-CoV-2 viral stock produced from a Colombian isolate (hCoV-19/Colombia/ANT-UdeA-200325-01/2020) (19).
AgNPs synthesis and characterization
AgNPs were synthesized by an electrochemical method using two Ag electrodes as anode and cathode, respectively. The Ag electrodes were placed into a thermostated electrolyzer equipped with a magnetic stirrer filled with water and two dissolved salts as the process initiators. Then the electrolysis was initiated, setting the correct voltage and current. A total of 16 parameters were controlled to ensure the AgNPs formation. The silver content in the synthesized AgNPs was determined by ICP-OES using a Thermo Scientific™ iCAP™ 7400 ICP-OES following the US EPA SW-846 Method 6010D. AgNPs stock solution was stored in a dark glass container at room temperature until use. The obtained AgNPs’ initial stock solution was used to prepare diluted solutions labeled as AgNPs-X, where AgNPs stand for silver nanoparticle solution, and X indicates the parts per million (ppm) concentration. Finally, diluted AgNPs-X solutions were stored in glass containers. The detailed process for obtaining the nanoparticles is under industrial protection of the Vitro company.
The obtained AgNPs solution was characterized by using ultraviolet-visible spectroscopy (UVVis) and transmission electron microscopy (TEM). UV-Vis was performed in an Orion AquaMate 7100 vis spectrophotometer in a range of 200-800 nm. The size and morphology of AgNPs were determined using a Tecnai F20 Super Twin transmission electron microscope, operating at an accelerating voltage of 200 kV. The average size of the individual particles was measured from TEM micrographs using the freeware ImageJ (20).
Cell viability assays
The viability of Vero E6 in the presence of AgNPs was evaluated using MTT (4,5-dimethylthiazol2-yl)-2,5-diphenyl tetrazolium bromide) assay. Briefly, Vero E6 were seeded at a cell density of 1 x 104 cells/well in 96-well plates and incubated for 24 hours at 37 ºC in a humidified 5% CO2 atmosphere. Then 150 µL/well of serial double dilutions (from 0.28 ppm to 0.008 ppm) of the initial AgNPs solution in cell culture medium were added to each well and incubated for 48 hours, at 37 ºC with 5% CO2. After incubation, the supernatants were removed, the cells were washed twice with phosphate buffered saline (PBS) (Lonza, Rockland, ME, USA), and 30 µL/well of the MTT reagent (Sigma-Aldrich) (2 mg/mL) were added. Then, the plates were incubated for 2 hours at 37 °C, with 5% CO2, protected from light.
Finally, formazan crystals were dissolved by adding 100 µL/well of pure DMSO. Plates were read in a multiscan GO spectrophotometer (Thermo) at 570 nm. The average absorbance of cells without treatment (treated only with cell culture medium) was considered 100% viability. Based on this control, the cell viability of each treated well was calculated. The wells with a viability of 80% or more after treatment were considered noncytotoxic. The 50% cytotoxic concentration (CC50) with 50% cytotoxicity was obtained by performing nonlinear regression and constructing a concentrationresponse curve (GraphPad Prism). For the MTT assay, two independent experiments with four replicates were performed (n = 8).
Antiviral assay
The antiviral activity of AgNPs against SARS-CoV-2 was evaluated with a pre-post strategy, adding treatment before and after the infection. Briefly, Vero E6 cells were seeded at a density of 1 × 104 cells/ well in 96-well plates and incubated for 24 hours, at 37 ºC with 5% CO2. After this, 50 µL/well of serial double dilutions of the initial AgNPs solution were added to the cell monolayers and incubated for 1 hour, at 37 ºC with 5% CO2. Then the pre-treatment was removed by discarding supernatants, and
cells were infected for 1 hour with 50 µL/well of SARS-CoV-2 stock at a multiplicity of infection (MOI) of 0.01 in DMEM supplemented with 2% FBS. After virus adsorption, the inoculum was removed and replaced by 170 µL of the same pretreatment dilutions. The plates were incubated for 48 hours, at 37
°C with 5% CO2. The cell culture supernatants were harvested and stored at -80 °C for virus titration by plaque assay.
The supernatant of infected cells without treatment was used as infection control. Chloroquine 100 µM has been used as positive inhibition control; two independent experiments with three replicates of each experiment were performed (n = 6).
Plaque assay for SARS-CoV-2 quantification
The reduction of PFU/mL of SARS-CoV-2 after treatment AgNPs solution dilutions was evaluated by plaque assay on Vero E6 cells. Briefly, 1 x 105 Vero E6 cells per well were seeded in 24-well plates for 24 hours, at 37 °C, with 5% CO2. Then, tenfold serial dilutions of the antiviral assay supernatants were added by duplicate on cell monolayers (200 µL per well). After one hour incubation, at 37 °C, with 5% CO2, the viral inoculum was removed, and 1 mL/well of semisolid medium (1.5% carboxymethylcellulose in DMEM 1X with 2% FBS and 1% penicillin-streptomycin) was added. The cells were incubated for four days at 37 °C, with 5 % CO2. Finally, the cells were washed twice with PBS and fixed/ stained with 500 µL of 4% formaldehyde and 1% crystal violet solution for 30 minutes. After two washes with PBS, the plaques were counted (21). The reduction in the viral titer after treatment compared to the infection control is expressed as inhibition percentage. Two independent experiments with two replicates were performed (n = 4).
Results
Characterization of AgNPs
A wide range of analytical methods is available to detect and characterize AgNPs (22-26). The choice of a specific method will depend on the type of information sought. Here, ICP-OES, UV-Vis, and TEM analytical techniques were used to obtain information about the concentration, and the size distribution of synthesized AgNPs.
The formation of AgNPs is confirmed by the appearance of the sharp, intense surface plasmon resonance (SPR) band in the range of 350-600 nm (27). From Figure 1A, AgNPs solution presents a sharp peak at 429 nm, indicating the formation of silver nanoparticles. SPRs depend on different factors such as size, shape, dielectric environment, and particles assemblies (27-29). It has been reported that the appearance of SPR under 500 nm indicates small particles with similar shapes (30). TEM analysis was performed to investigate the morphology and size of synthesized AgNPs, to the initial solution. The results are shown in Figure 1B-C.
Cytotoxicity of AgNPs solution on Vero E6
The effect of AgNPs solutions on cell viability is shown in Figure 2A. After 48 hours of treatment, Vero E6 cells exhibited viabilities of 6.4%, 6.5% and 10% for AgNPs solution at 0.28, 0.14 and 0.07 ppm, respectively. In contrast, for more diluted concentrations of AgNPs, the cell viability was 100%. Based on cytotoxicity results, the antiviral activity of the AgNPs solution was evaluated from 0.03 ppm. From the cytotoxic assay, a CC50 of 0.062 ppm was calculated for AgNPs solution.
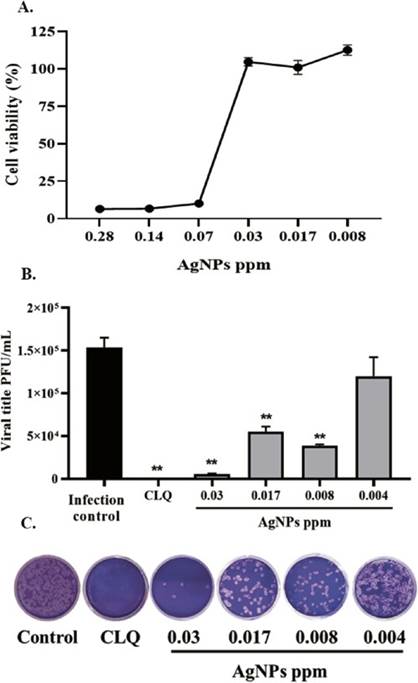
A) AgNPs solution cytotoxicity on Vero E6 cells. Data are shown as Mean ± SEM. B) AgNPs solution antiviral activity against SARS-CoV-2 (n = 4). Positive control of viral inhibition: Chloroquine (CLQ). The asterisks represent the statistically significant differences compared with the untreated control (*p ≤ 0.05, **p ≤ 0.01; Mann-Whitney U test). C) Representative figure of SARS-CoV-2 plaque assay on SARS-CoV2 infected Vero E6 cells
Antiviral effect of AgNPs solutions on SARS-CoV-2 infected cells
The antiviral effect of AgNPs solution was evaluated on SARS-CoV-2 infected cells, in the concentrations previously determined, according to cell viability assay and CC50 results. The evaluated AgNPs solution presented a significant reduction of SARS-CoV-2 viral titer after a pre-post treatment strategy with inhibition of 96.5% (p = 0.009), 64.13% (p = 0.009) and 74.72% (p = 0.009) at 0.03, 0.017, and 0.008 ppm respectively, compared to infection control (Figure 2B-C).
Nonlinear regression was done to determine the EC50 and selectivity index (SI) of the AgNPs solution. AgNPs solution shown an EC50 of 0.005 ppm and a selectivity index of 12.4, indicating that AgNPs solution has high selectivity (32).
Discussion
Silver nanoparticles have drawn the attention of the scientific community due to their proven effective antiviral action. Two key factors -particle size and concentrationhave shown to be of paramount importance in vitro and in vivo studies (33,34). The AgNPs size has been related to the capability of AgNPs to interact with the viral proteins. Anti-SARS-CoV-2 activity has been reached for AgNPs diameters between 2 and 15 nm (35,36). The AgNPs prepared here by the electrochemical method generated silver nanoparticles with diameter sizes ranging between 2.6 to 30 nm, with an average size of 6.2 nm, which falls in the optimal antiviral activity reported by others. The cytotoxic effect was observed in 0.07-0.28 ppm concentrations, but not in 0.03, 0.017, and 0.008 ppm solutions. AgNPs exhibited a significant reduction of SARS-CoV-2 viral titer after a pre-post treatment strategy with inhibition of 96.5%, 64.13% and 74.72% at 0.03, 0.017, and 0.008 ppm, respectively.
A primary concern related to AgNPs applications is their toxicity due to the increased oxidative stress, apoptosis, and genotoxicity caused in cells upon exposure. Several studies have concluded that silver nanoparticles toxicity depends on the cell type, particle size, and concentration (34,37). Recently, Sundararaj et al. (36) reported the cytotoxic effect of AgNPs on mammalian cells at 20 ppm and above. They also observed that even AgNPs with a diameter size of 2 nm could exhibit toxicity even at 2 ppm. Here we found cytotoxic effects at lower values (0.07-0.28 ppm), indicating that caution should be exercised when preparing AgNPs formulations for antiviral purposes.
Virucidal agents act directly and rapidly by lysing viral membranes or by binding to virus envelope proteins. The mechanism of action of AgNPs as antiviral and virucidal has been studied against several enveloped viruses. The antiviral activity of AgNPs against other viruses such as HIV, hepatitis B virus, herpes simplex virus, respiratory syncytial virus, and monkeypox virus has been demonstrated (38,39).
In accordance with our results, AgNPs with different surface modifications and particle size have shown virucidal effects against SARS-CoV-2 in vitro (40). AgNPs can affect the viral cycle during early or late viral stages. Previous studies suggest that AgNPs can bind to viral proteins involved in cell receptor binding. Early replication steps inhibition could be related to decreasing the viral transmission, which could directly impact the incidence of SARS-CoV-2. Further studies are required to assess this hypothesis.
In addition to this report, additional assays evaluating the activity of AgNPs against the SARSCoV-2 variant are also required, especially when considering the cumulative mutations and their ability to evade the immune response. For instance, previous reports have shown differences in the antiviral activity of atorvastatin against ancestral B1 strain (D614G), Delta and Mu variants (41).
Interestingly, AgNPs preferentially bind to viral surface proteins rich in sulfhydryl groups. As disulfide bonds are essential for binding SARS-CoV-2 spike protein to the angiotensin-converting enzyme-2 (ACE2), AgNPs could prevent recognizing the target cell and subsequent SARS-CoV-2 entry by direct interaction with viral proteins (36). AgNPs are potentially absorbed by cellular processes such as endocytosis and micropinocytosis (42). Inside the cell, they can inhibit the virus replication by interacting with viral nucleic acids (43).
Antiviral activity of AgNPs against SARS-CoV-2 was described in vivo in a study performed with healthcare personnel by using mouthwash and nose rinse. AgNPs prevented SARS-CoV2 infection significantly (1.8% [2 participants out of 114]), compared with the control group which received
conventional mouthwash and nose rinse without AgNPs (28.2% [33 participants out of 117]) (18). However, the interaction of AgNPs with viruses remains undetermined and although there is significant evidence regarding the antiviral effect of AgNPs, further investigations evaluating their cytotoxic effect in different cell lines, animal models, and clinical assays are required to determine the potential use of AgNPs in human medicine, as well as the dose and the route of AgNPs administration, specifically in the treatment of SARS-CoV-2 infection.