1. INTRODUCTION
Scaffolds are composed by nanofibers, they have different topographic characteristics such as high porosity, large surface area per unit mass (for example, nanofibers with 100 nm in diameter have a specific surface area of 1000 m2/g), high permeability, as well as the ability to mimic the extracellular matrix (ECM) of tissues both chemically and mechanically [1] . Nanofibers are made up of one or more polymers, they can carry embedded nanoparticles, becoming a nanocomposite material. These are widely studied for various applications such as filters, wound healing and healing dressings, scaffolds in tissue engineering, controlled drug release, immobilization of enzymes, biosensors, power generation, protective clothing, nanotextiles for medical use applied to the reduction of viruses and bacteria, membranes, and cosmetic wipes [2]. The techniques commonly used for producing nanofibers in general include drawing, template synthesis, phase separation, self-assembly and electrospinning [3]-[5] .
Electrospinning ( Figure 1A), is a method to produce 2D or 3D nanofibers, from a natural or synthetic polymer solution, where a high voltage is applied to generate aligned or random fibers with diameters from nanometers to micrometers [6] - [8] . This allows to find scaffolds with unique characteristics, such as a very large surface area in relation to volume, high porosity, a morphology similar at natural extracellular matrix (ECM), interconnected pores and superior mechanical performance to promote regeneration, in tissue engineering through cell adhesion, migration, and proliferation, to create new artificial tissues as substitutes [6], [9], [10] .
Electrospinning allows to create nanofibers with diameters below 500 nm [11]. Electrospinning has proven to be simple, versatile, cost-effective, and reproducible [2]. A typical electrospinning, consists of three main components: (1) a high voltage source, which guarantees a continuous electric field with a positive and a negative polarity; (2) an infusion pump, adjusted to control the feed rate of the polymer solution contained in a syringe; and (3) a metal collector (usually a stationary aluminum plate or a rotating cylinder) where the resulting fibers are deposited [12], [13]. Many parameters are set up during the electrospinning process, including the properties of the solution (polymer concentration, viscosity, electrical conductivity, surface tension, and dielectric properties). In addition to processing parameters such as voltage, speed of flow, the distance between the needle and the collector plate. Environmental parameters such as humidity and temperature. Those parameters directly affect the formation and structure of the fibers and can facilitate the presence of drop defects [2].
A brief history of electrospinning technique, Anton Formhals filed several patents between the years 1934 and 1944, the first patent in the United States that described the electrospinning operation in 1934, to produce cellulose acetate filaments using an electrostatic force of 57 kV and in 1944 he reported a patent with improvements towards the commercialization of electrospinning, in the mobile collector to collect textile yarns in a stretched condition, such as that of a rotating drum in conventional spinning [14]. In 1969 Sir Geoffrey Ingram Taylor contributed significantly to the theory of electrospinning, he mathematically modeled the shape of the cone formed by the drop of fluid when it is exposed to an electric field. This shape that the drop takes is now called the Taylor cone [14], [15]
Despite these early discoveries, the process was not used commercially with great success until the early 1990s, when the term "electrospinning" was coined from the previously used term "electrostatic spinning". Around 30 patents were issued on the electrospinning technique, gaining popularity thanks to a growing interest in nanotechnology and due to improvements in characterization techniques and the ability to manufacture complex scaffolds. In 1996, Reneker and Chun demonstrated the manufacture of nanofibers from various polymers applied to different areas of science and industry [15]. In recent years, some companies such as Inovenso Inc, Elmarco, Bioinicia, InoCURE, The Electrospinning Company Ltd, Electrospinning BioInnovations Group, IME Medical Electrospinning, Nanofiber Solutions, The Stellenbosch Nanofiber Company, eSpin Technologies, NanoTechnics, KATO Tech, more than 200 universities and research institutes are actively involved in investigations related to electrospinning, nanofiber creation and their applications, while companies such as Donaldson Company and Freudenberg obtain their air filtration products by electrospinning [3], [6] .
Some current applications of electrospinning are in biomedical areas as tissue engineering, drug delivery, wound dressings, catalyst, and enzyme carriers. Industrial applications such as cosmetics, composite reinforcement, air filter, affinity membranes and recovery of metal ions in water, smart textiles and protective clothing, battery /cell capacitor, sensor, catalyst [5].
The traditional electrospinning apparatus had been modified to explore new possibilities and improve the nanofiber properties, because 2D scaffolds show insufficient cellular infiltration and high packing density. Other techniques derived from electrospinning have been needleless electrospinning [17], multineedle electrospinning [18], coaxial electrospinning [19], melt electrospinning [20], bubble electrospinning [3], blow electrospinning [21], and wet electrospinning [22]. Some techniques such as melt electrospinning and wet electrospinning are inspired in traditional fabrication techniques of woven fabrics (melt spinning and wet spinning) respectively.
Wet spinning, (Figure 1B) is the oldest textile technique used to produce synthetic polymeric fibers, such as nylon (polyamide), spandex, rayon, aramid, and acrylic. It is based on an extrusion with the air of a very viscous polymer solution directly into a coagulation bath usually cold water or in an organic solvent, or in a weak inorganic acid, to form a filament emerge from the solution, then, they stretch and solidifies as continuous fiber with a final diameter on the order of micrometers, these fibers are washed, dried and finally collected in a rotor [23], [24]. Wet spinning has low productivity of fibers, compared to melt spinning and dry spinning techniques. To improve this technique, the filaments need to wash to remove impurities of solvent [24]. James Kay in 1825 invented of wet spinning of linen fibers in the industrial revolution. Later, Du Pont (USA) patented the first process of wet spinning and produced fibers registered under the name "Orlon" [25]. In 1968, was performed acrylic fibers into gelled solution by wet spinning also known as coagulation process [24].
Currently, Russia and China, produce fine linen threads.
Wet electrospinning emerged as a combination of electrospinning and wet spinning for the laboratory-level production of 3D structure of nanofibrous (Figure 1C). This technique consists of a polymeric solution within a syringe connected to a pump, that ejects with a flow rate programmed the solution and with high voltage it creates fibers. These are immersed in a coagulation bath as a collector. The liquid bath consists of a non-solvent for the polymer solution (such as methanol, ethanol), are commonly used due to their low surface energy. In this coagulation bath, the solution solidifies to form long fibers with diameters between 30 to 600 μm, depending on the needle diameter, polymer composition, and flow rate [23]. As well, the coagulation bath, can be used to perform a post-modification of the scaffold as a surface coating, functionalization and crosslinking in a single-step [22]. The low surface tension of the solvents used as a liquid bath allows the fibers to sink, it has influences in thickness, packing, dispersion, and distribution of fibers, and acquired a porous structure, bulk density and low apparent density of the fiber decreases [16], [22]. For example, water has a surface tension of 70-72.58 mN/m hinders the fibers to dip, thus produces dense and less porous fibers, while, Butyl alcohol has a surface tension of 20.61 mN/m, it allows the fibers to sink into the coagulation bath, organizing themselves into a 3D structure which produces fibers with less apparent density and more porous [22], [26].
Nevertheless, an additional molding and freeze-dried process after wet electrospinning is usually required to retain the 3D shape [4], [22], [23], [27]. The most important parameters in wet electrospinning are non-solvent coagulation bath, the polymer concentration and molecular weight, the presence of additives and the solution feed rate [23].
The high pore size of 3D scaffolds, development by wet electrospinning varies among 250-500 μm, measured by Branauer Emmet Teller (BET) porosimetry (gas absorption) or by processing micrograph images in software such as Image J, this characteristic allow improves cell adhesion, infiltration, and proliferation inside of the scaffolds for tissue engineering applications, in compared with 2D scaffolds which exhibit smaller pores [22], [28]. This technique allows to control the size of 3D scaffold in terms of the width and height by deposition time, and the parameters as solution concentration, voltage, flow rate, distance, coagulant with low surface tension, which allows to produce small fiber diameter between nano-micro scale, wet electrospinning technique is reproducible and inexpensive [22].
Polymers with low viscosity that cannot be spun by other procedures, it can be used by wet electrospinning to create fibrous scaffolds [3], [27]. Natural polymers as chitosan and collagen that it is difficult to process to form scaffolds by other techniques such as melt electrospinning due to their low thermal degradation can be used by wet electrospinning [23]. It is necessary improve mass production of wet electrospinning for industrial applications [22].
Coaxial configuration of syringes (Figure 1D) has been used for loaded drug, encapsulation, and drug delivery in wet electrospinning in a core-shell structure of bioactive compounds, including antibiotics, proteins, growth factors and genes [23].
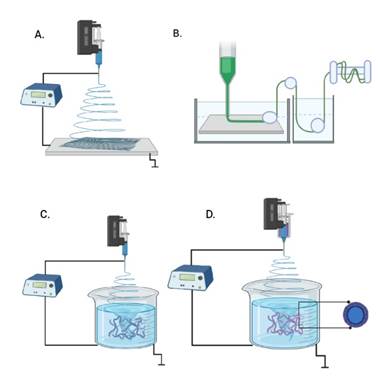
Source: Authors based on: [22].
Figure 1 Techniques for producing fibers. A. Electrospinning. B. Wet spinning. C. Wet electrospinning. D. Coaxial Wet electrospinning
Wet electrospinning also known as wet electrospun, dry-wet electrospinning, liquid assisted electrospinning and immersion electrospinning (I-ESP), was first described by Yang, W et.al. 2005 of Donghua University, China, which created ultrafine Poly (m-phenylene isophthalamide (PMIA) fibers in water as ionic liquid [29]. Nevertheless, this technique was not patented until 2010 in the United States, by the register US34903110P was granted to Yi Hong, Nicholas J. Amoroso, Kazuro Lee Fujimoto, Ryotaro Hashizume and William R. Wagner of the University of Pittsburgh, who created a Poly (ester urethane) urea (ePEUU) scaffold to repair abdominal wall. Since 2019 this patent has been recognized under the register US20190046689A1[30].
In our knowledge, a few authors have reviewed the wet electrospinning technique, Puppi et. al. 2017, presented a mini-review where explain how wet electrospinning can be modified by computer and could be used to additive manufacture (AM), to expand their productivity from lab scale to industrial scale [23]. Taskin et.al. 2020, presented the advantages of 3D scaffolds morphology, explain how chemical functionalization in situ can improve the properties of scaffolds, and how obtain nanotopographical alterations in 3D scaffolds using liquid bath as phase separation in one step to produce porous fibers [22]. So far, there is no review that includes the current applications of wet electrospinning, which allows to have a greater worldview of the possible future work of researchers in this area. In this work, we present a review of the principal applications of wet electrospinning, we select papers between 2017 to 2021, in the beginning tissue engineering will be addressed such as cardiac, cartilage, hepatic, wound dressings and skin tissue, neural, bone, and skeletal muscle, drug delivery. Continuously, industrial applications will be covered between them water purification and air filters, energetic applications and biomedical sensors and flame retardants.
2. WET ELECTROSPINNING APPLICATIONS
Wet electrospinning has applications in cardiac, cartilage, hepatic, wound dressings, and skin tissue, neural, bone, and skeletal muscle for tissue engineering, as well as other industrial applications such as water purification and air filter, biomedical sensors and flame retardants.
2.1 Tissue engineering
Tissue engineering for regenerative medicine aims to develop scaffolds from natural or synthetic polymer biomaterials, this discipline emphasizes the critical role of structure and biomechanics in scaffolds to seed cells and use growth factors, and then with this graft replacement different organs. 3D scaffolds are biocompatible, biodegradable, and biomimetic extracellular matrix (ECM) of natural tissues and its presets macro porous allows differentiation and proliferation of cells inside scaffolds, without the steric hindrance of cell motility and provides structural support similar to tissue objective [31], [32].
2.1.1 Cardiac or soft tissue engineering
Wet electrospinning has been used to create soft tissue engineering 3D scaffolds, these must possess good mechanical properties, to support cardiomyocytes and stem cells functions.
The ideal polymers for this application are biodegradable, with structural stability and thermoplastic elastomers, with elastic modulus (E) in the range 0.1-100 MPa, these must be biomimetic depending on the cardiac tissue to be replaced, which enable them to maintain their integrity to endure cyclic deformation and to transfer mechanical stress similar to cardiac muscle and blood vessels, while it allows cell growth [33]. Helically coiled scaffolds (HCS) can mimic the morphology and behavior of the heart muscle perimysium composed of microscale coiled fibers. These scaffolds can mimic the morphology of the heart muscle, provide superior adhesion, proliferation and deep cell infiltration due to their high porosity (Table 1) [33]-[36].
Sonseca et. al. 2020, developed architectures of 3D helically coiled scaffolds (HCS) from segmented co-polyester of poly (butylene succinate-co-dilinoleic succinate) (PBS-DLS) (70:30) at 20 % w/v in the solvent chloroform-methanol (7:3) vol, and for the coagulant solution they used (water, ethanol, and methanol), with surface tension (72.86, 22.10, 22.50 mN/m, respectively). In water, the scaffolds floated and formed a 2D structure. Whereas scaffolds in ethanol and methanol show no difference in the morphology. The authors affirm that, the low surface tension in coagulant solution allows a low packaged structure and avoid the fibers from accumulating upwards. By adjusting the wet electrospinning parameters, such as flow rate and voltage, different diameters, and morphologies, flat ribbon-like and coil-like fibers similar to heart perimysium, can be obtained. It was found that, 3D scaffolds have more open pores, better mechanical properties and show 2 times higher proliferation than 2D scaffolds.
The scaffolds were evaluated by seeding L929 murine fibroblasts, which showed no cytotoxicity [33].
Krishnamoorthi et. al. 2020, fabricated a 3D scaffold of Poly lactide-co-glycolide (PLGA) at 8 % w/v in chloroform: dimethylformamide (7:3) vol, as a solvent, and isopropyl alcohol/ distilled water (7:3) ratio, as coagulant, and 0.05 % F108 surfactant to reduce the surface tension of water. This scaffold induced spontaneous differentiation of human stem cells, maintained viability, the authors highlight the importance of bioactivity in the scaffolds to resemble the extracellular matrix and allow the differentiation of stem cells from cardiac tissue and the measurement of proteins and specific markers that indicate the formation of new tissue [35].
Majidi et. al. 2018, fabricated a 3D scaffold macroporous, sodium alginate (Mw = 396 kDa)/poly (ethylene oxide) (PEO) (Mw = 900 kDa) at 2, 4, 5 % w/v, and gelatin from cold water fish skin hydrogel (Mw = 60 kDa), in deionized water as a solvent, and they used water: ethanol (1:5) ratio, as coagulant with 2 % w/v of calcium chloride and Pluronic F-127 surfactant, scaffold was freeze-drying. The authors indicated the requirement of a convergence between biological needs and the development of biomaterials that meet these requirements, for example 3D scaffolds mimic the extracellular matrix and in future works it is expected that a total integration with the native tissue can be achieved. The use of the Pluronic F-127 surfactant allows to reduce the surface tension of the water, improving the electrospinning of the alginate, the calcium chloride improved the coagulation and the morphology of the fibers, the low surface tension of the ethanol helped to create a macroporous and fiber structure not compact. Due to the macropores, the scaffolds improved the adhesion in human IPSC-derived ventricular cardio myocytes [34].
Liu et. al. 2017, fabricated scaffolds of Raw pulpis with 87 % alpha-cellulose 2 % and 3 % w/v, with ibuprofen 6 % wt, in 1-Butyl-3-methylimidazolium chloride [BMIM]C as a solvent, and water as coagulant, scaffolds were cultured with mouse HL-1 cardio myocytes, and these substrates exhibited cellular viability, the authors performed an analysis of the morphology of the fibers with respect to the concentration of the cellulose at 2 % wt some fibers were fused, while at 3 % wt the areas of fiber fusion disappeared, the ibuprofen remained distributed on the surface from the scaffold or trapped within its pores [36].
2.1.2 Cartilage tissue engineering
Cartilage has limited self-repair due to lack of blood or nerve supply and the low proliferation rate of chondrocytes [37]. Wet electrospinning allows to create 3D scaffolds for cartilage tissue engineering, with the use of natural and synthetic polymers. That offers similar anisotropic network organization of native natural collagen fibers and gives stress resistance and low friction at mechanical movements, reducing the possibility of osteoarthritis and risks associated of hypertrophy and ossification. The objective of these scaffolds is to restore the mobility function and protecting the chondrocytes, and does not shrink over time, ideally, which generates scar tissue [31], [38]. To regulate chondrogenic differentiation of stem cells is necessary the addition of functionalized peptides and growth factors in the polymer solution, to stimulate the cartilage formation [31], [38].
Natural polymers can be blended with synthetic polymers to improve mechanical strength, porous scaffolds are better for chondrocyte infiltration [39]. The knee cartilage withstands a maximum compressive force of 0.84 to 3 MPa in a person weighing 70 kg [31].
The use of hydrogels is popular due to their similarity between its hydrated structures and the hydrophilic environment of the cartilaginous ECM, new approach is about the combination of hydrogels with fibers as reinforcement that improves tensile strength [37], [39].
Li et. al. 2020, developed a meniscus substitute by wet electrospinning with fibroin of Bombyx mori cocoons, polycaprolactone (PCL) (Mw: 80 kDa) (1:4) ratio, and strontium chloride (SrCl2) (20 mmol/L) in hexafluoro isopropanol (HFIP) and water (90:10) as solvent, collected in 7 ml of anhydrous ethanol as coagulant, their pore size varied between (10-20 μm) and mechanical support (61.6 ± 2.9) MPa for traction and (0.11 ± 0.03) MPa for compression). The addition of Sr to the meniscus 3D scaffold strongly stimulated ECM formation in vitro, enabled type II collagen expression and attenuated cartilage degeneration in a rabbit model with meniscectomy. The thickness of the scaffold was regulated by adjusting the deposition time and the pore size was regulated by the concentration of the solution. In future work, the authors propose to add chemo-attractants or growth factors to the scaffolds for functional regeneration of the meniscus and to delay the development of osteoarthritis [38].
Rafiei et.al. 2020, prepared the protein bovine serum albumin (BSA) 10 mg/ml with distilled water as solvent, and PCL (Mw: 80.000 Da) 10-15 % w/v with chloroform/ dimethylformamide (DMF) 70:30 v/ v as solvent, collected in water/ ethanol (1:9 v/v) as coagulant in a beaker of 3 cm deep, by coaxial wet electrospinning to develop 3D scaffolds with a core-shell structure for cartilage tissue replacement. The high viscosity of the PCL solution, coaxial needle gauge and applied voltage produced relatively large fiber diameters.
The porosity of 3D scaffolds could be controlled by density, hence by compression. By increasing the compaction of the scaffolds by decreasing the pore size, which causes the Young's modulus to increase (0.3-5 MPa). Scaffold was biocompatible with human fibroblast cells [31].
Gunes et. al. 2020, fabricated 3D multi-layered scaffolds of poly (3-hydroxybutyrate-co-3-hydroxyvalerate) (PHBV) (Mw: 80 kDa) at 3 % w/v, and Benzyl triethylammonium chloride (BTEAC) 0.2 % w/v, with chloroform as solvent, collected in ethanol-distilled water mixture at (9:1) volume ratio and carboxymethyl chitosan (CMC) - fibroin of Bombyx mori cocoons hydrogel. The scaffolds mimicked the hydrated ECM structure of cartilage through an interconnected microporous structure, increased compressive strength (207 +/- 45 kPa), that resulted good cartilage substitute due to promotes chondrogenic differentiation of bone marrow mesenchymal stem cells (BMSCs) [37]. These scaffolds showed improved mechanical properties in compression, and imitate EMC of cartilage both in vitro with chondrocytes and in vivo with rabbits (Table 2) [31], [37]-[39].
2.1.3 Hepatic tissue engineering
Due to the few liver donors, the development of 3D grafts that can maintain the functionality and viability of the hepatocytes has been promoted due to their similarity of random fibers and porosity with the extracellular matrix of the liver. Proteins such as collagen I and fibronectin present in the natural microenvironment enable the functions and interactions of hepatocytes, gene expression, and cell phenotype [40], [41]. The electrospinning technique has the disadvantage that the high density of fibers prevents cellular infiltration in the scaffold, while wet electrospinning allows the production of less dense and more porous fibers, which facilitates the insertion of the hepatocytes inside the 3D scaffold [40], [41]. In hepatic tissue engineering have been used poly (lactic-co-glycolic acid) (PLGA), Collagen-I, and fibronectin in culture with hepatocytes, these scaffolds improved albumin secretion and increase the expression of gene CYP3A4 (Table 3) [40], [41].
Das et. al. 2020, fabricated 3D scaffolds highly porous of poly (lactic-co-glycolic acid) (PLGA) (80:20) with chloroform: dimethylformamide (DMF) (7:3) ratio as solvent, collected in isopropyl alcohol and deionized water 7:3 (v: v) as coagulant. Scaffold was modified with collagen-I and plasma fibronectin (3:1) ratio. Protein-containing scaffolds showed better Huh75 cell viability, improved the cell microenvironment, provided efficient chemisorption, and increased the albumin secretion and CYP3A4 - CYP3A7 expression in 28 days of culture [40].
Brown et. al. 2018, developed a 3D scaffold of poly (L-lactide-co-glycolide) (PLGA) with chloroform: dimethylformamide (DMF) (7:3) ratio as solvent, collected in isopropyl alcohol and deionized water 7:3 (v: v), with 0.05 % wt Pluronic F-108 used as surfactant. Scaffold was modified with collagen type I. 3D scaffold was cultured per 2 weeks with primary human hepatocytes, this guide 10 times more secretion of albumin and increase the transcription of hepatocyte specific CYP450 genes (CYP3A4, 3.5 times and CYP2C9, 3 times) in comparing to scaffolds without protein treatment. Wet electrospinning produces scaffolds with larger pore size compared to traditional electrospinning which enables incorporation of hepatocytes into the matrix [41].
2.1.4 Wound dressings and skin tissue engineering
Chronic wounds represent a problem due to these could not cicatrize and the closure of these is not achieved, which can cause infections. An ideal scaffold should imitate the natural ECM, providing signage to cells and growth factors to the site of injury and cover the wound bed, which facilitates angiogenesis, collagen deposition and granulation tissue formation, while improving the healing process [42]. Skin defects can occur due to burns, soft tissue trauma, and diseases that lead to necrosis, for defects that exceed 60 % of the skin due to deep burns, it is difficult to find an autologous donor site. Whence, scaffolds that can imitate the natural ECM of collagen fibers and elastin, to produce an environment that facilitates angiogenesis, promote cicatrization are preferred, and allows increased surface area for cell attachment. Additionally, a high porosity is desirable for cellular infiltration [43]. The fibroblasts and stem cells on in vitro culture with scaffolds showed non-cytotoxicity, cell infiltration, migration and proliferation improve the wound healing (Table 4) [42]-[48].
Table 4 Recent studies in wet electrospinning for wound dressings and skin tissue engineering
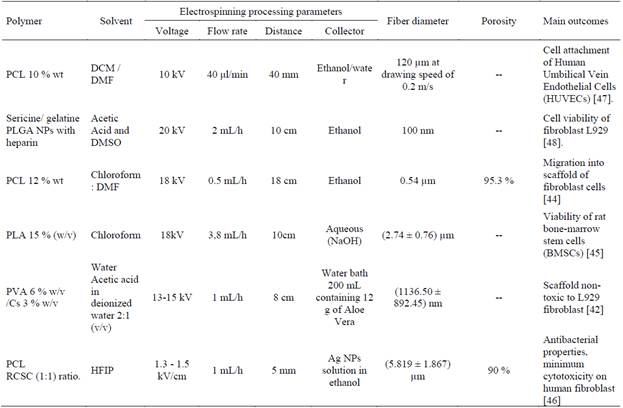
Source: Authors
Jiang et. al. 2021, fabricated sandwich scaffold of polycaprolactone (PCL) (Mw: 80.000 Da), with Dichloromethane (DCM): Dimethylformamide (DMF) (2:1) (v: v) as solvent, collected in ethanol/water as coagulant. Differences in the surface tension of water (72.8 mN/m) and ethanol (22.1 mN/m) produced fibers with different characteristics. Due to the high surface tension of water, the net-like fibers cannot break the hydrogen bond at the water surface and floated, they broke easily when pulled out of the water, due to the high adhesive force between the water molecules and the fibers. The fibers formed a yarn structure, immersed in the ethanol and formed into a continuous bundle upon removal from the ethanol bath. When the ethanol/water ratio was less than (28.52 mN/m), a continuous fiber bundle was obtained. Scaffold was inspired in crochet textile technique to imitate collagen porous fibers, using wet electrospinning with drawing modification in coagulation bath. It allows to obtain different nanofibers diameter according to their drawing speed, this sandwich morphology allowed cell attachment of Human Umbilical Vein Endothelial Cells (HUVECs) [47].
Akolpoğlu Başaran et. al. 2021, development 3D scaffold of sericine/Type A gelatin (1⁄2 v: v) (20 % wt) with acetic acid and dimethyl sulfoxide (DMSO) as solvent, collected in ethanol as coagulant. 3D scaffolds were supplemented with PLGA (Mw:7-14 kDa) nanoparticles loaded with heparin, the cell viability of fibroblast L929 increased with the content of heparin at 72 hours of in vitro culture. The presence of higher serine-containing nanoparticles in the scaffold significantly increased the proliferation of L929 cells by the fifth day of incubation. Heparin stimulates fibroblast cell proliferation. The authors emphasize the need for further investigation of the wound healing/skin regeneration potential of the produced materials [48].
Jing et. al. 2019, developed scaffolds of Polycaprolactone (PCL) (Mw: 80.000 g/mol) 12 % wt, with chloroform: Dimethylformamide (DMF) (6:4) as solvent, collected in ethanol bath as coagulant saturated with carbon dioxide (CO2) and introduced into a water bath, in which they expanded due to the escape of CO2. The porosity of the 3D scaffold was 95.3 %, much higher than that of the 2D scaffold. The 3D scaffold mimicked the ECM through porosity and surface area and favored the growth of 3T3 human fibroblasts, with enhanced cell adhesion and increased cell viability [44].
Ghorbani et. al. 2018, prepared 3D high porous scaffolds of polylactic acid (PLA) (Mw: 160.000 Da) 15 % (w/v) with chloroform as solvent, collected in aqueous solution of sodium hydroxide (NaOH, pH 13) as coagulant. 3D scaffolds of PLA highly porous with a high surface area allows appropriate gas exchange and exudate absorption. The in vitro and in vivo results suggest that PLA 3D scaffold seeded with rat bone-marrow derived mesenchymal stem cells (BMSCs), was nontoxic and biocompatible for wound repair [45].
Naseri-Nosar et. al. 2017, developed a 3D scaffold by wet electrospinning of recombinant human erythropoietin/aloe Vera gel-releasing polyvinyl alcohol (PVA) 6 % w/v in water /Chitosan (Cs) 3 % w/v in acetic acid in deionized water 2:1 (v/v), collected in water bath 200 mL containing 12 g of Aloe Vera. Aloe Vera has anti-inflammatory and immunomodulatory effects on wound healing. It also increases fibroblast growth and activity and increases the level of collagen in the wound. In addition, it increases the content of hyaluronic acid and dermatan sulfate in the granulation tissue. RhEpo caused considerable angiogenesis in the treated wounds, accelerated re-epithelialization and shortened wound inflammation. 3D scaffold was not toxic with L929 fibroblasts and improved the wound healing in Wistar rats in comparing with sterile gauze [42].
Zhang et. al. 2017, fabricated 3D scaffold by wet electrospinning with ranachensinensis skin collagen (RCSC) and polycaprolactone (PCL) (Mw:52.000 Da) 10 % wt (1:1) ratio in 1,1,1,3,3,3, -hexafluoro-2- propanol (HFIP) as solvent, collected in silver nanoparticles (Ag NPs) solution in ethanol 25 mg/L, 50 mg/L, 75 mg/L, and 100 mg/L. By increasing the concentration of the silver nanoparticles, the uniformity of the fibers was reduced, generating larger fiber diameters and increased pore sizes. 3D scaffolds had a porosity of 90 %, reinforces antibacterial properties and present minimum cytotoxicity in human fibroblast [46].
2.1.5 Neural tissue engineering
Nerve injuries caused by physical trauma, diseases as Alzheimer, Huntington, Parkinson spinal muscular atrophy (SMA), or genetic problems, result in the loss of motor and sensory functions, which causes disability. The objective of neural tissue engineering is to repair and to conduct of guide the neural alienation, further direct axonal extension, and neural growth.
By manufacturing 3D cylindrical scaffolds from biodegradable and biocompatible polymers that create an environment that mimetic the structure and components of autologous nerve [49]-[53]. Carbon nanotubes (CNT) and conductive polymers such as polyaniline have been employed as a conductor material to improve neural signaling [50], [52]. These scaffolds cultured in vitro with neurons, rat Schwann cells and stem cells, showed mimicking the epineurium layer protected nerve, supported the growth and improvement cells differentiation and proliferation into the neuron, scaffolds enhanced the regeneration of nerve (Table 5) [49]-[55].
Garrudo et. al. 2021, achieved differentiation of REN-VM cell into neural cells (neurons and astrocytes) on polycaprolactone (PCL) (Mw: 80.000 Da) 13 % (w/v) with trifluoroethanol (TFE) as solvent, collected in water as coagulant. 3D scaffolds with glycosaminoglycans (GAG), as hyaluronic acid (HA), chondroitin sulfate and heparan sulfate, allows the creation of complex structures ECM, such as peripheral networks. Improving the design of electroconductive scaffolds for neural tissue engineering applications. Disease mechanisms can be studied, and new drugs tested, for the development of implants for transplantation in patients with neurological diseases [53].
Ranjan et. al.2020, encapsulated pluripotent stem cell (IPSC) in 3D scaffold of poly (lactic-co-glycolic acid) (PLGA) 10 % wt with chloroform: dimethylformamide (DMF) 7:3 ratio as solvent, collected in ethanol. Although, 3D scaffold reduced cell proliferation, accelerates neuronal differentiation. The ethanol bath increased the dispersion of the fibers and reduced their binding. Pore size decreased with increasing solution concentration. The scaffolds had large, interconnected pores with lower fiber packing densities compared to 2D fiber mats, which allowed cells to easily infiltrate inside them [55].
Wang et. al. 2019, developed a 3D scaffold of polycaprolactone (PCL) (Mw: 80.000 Da), silk fibroin (SF), and carbon nanotubes (CNTs) (8:2:0.1 ratio) with hexafluoro isopropanol (HFIP) as solvent, collected in distilled water/ ethanol (8:2 v/v) as coagulant. The 3D scaffolds exhibited biocompatibility with PC12 cells, provided a suitable microenvironment for improved alignment and extension, hydrogel shell mimicking the epineuron layer protected their organization [49].
Ghorbani et. al. 2018, seeded human Wharton jelly-derived mesenchymal stem cells on 3D conductive scaffolds of polylactic acid (PLA) 10, 13, 15, 17, 20 % w/v with chloroform as solvent, collected in Sodium hydroxide (NaOH) and coated with natural polymers including alginate and gelatin, followed by a multi-wall carbon nanotube (MWCNT) coating, this scaffold supported the growth and improvement hMSCs cell differentiation, they expressed neuronal markers: Nestin and Neuro-D1 [50].
Farzamfar et. al. 2018, produced 3D scaffold of cellulose acetate (CA) and gelatin (Gel) 1:1 ratio, 8 % w/v with acetic acid and glutaraldehyde 25 % (w/v) as solvent, collected in water/ethanol (3:7) v/v and gabapentin (GBP) 3, 6, 12 % w/v. Scaffold shown to be compatible with Primary rat Schwann cells (SCs), in the in vivo study the scaffold obtained from 6 % (w/v) GBP enhanced the regeneration of created injury in a sciatic nerve defect model in Wistar rats [51].
Naseri-Nosar et. al. 2017, developed a biodegradable 3D drug-loaded scaffolds with the core-shell structure with polylactic acid (PLA) 10 % wt was dissolved in 1, 4-dioxane as core /cellulose acetate (CA) 8 % wt was dissolved in acetone as shell / citalopram-loaded gelatin nanocarriers (CGNs), 3D scaffolds was collected in water as coagulant, they shown biodegradability and cytocompatibility with rat Schwann cells, in vivo study in a sciatic nerve defect revealed that scaffolding with citalopram enhanced lesion regeneration [54].
Zamani et. al. 2017, fabricates electrical excitable 3D scaffolds of polyaniline (PANI) (Mw: 50.000 Da)/ poly (lactic-co-glycolic acid) (PLGA) 3 % (w/v) and polycaprolactone (PCL)(Mw: 80.000 Da) scaffolds with chloroform: dimethylformamide (70:30) as solvent, collected in water as coagulant, 3D scaffolds were biocompatible and effective to electrical stimulation The authors emphasize that future study will focus on functional conductive scaffolds in the presence of electrical stimulation in nerve cells. 3D scaffold was effective to promote A-172 cell proliferation for spinal cord regeneration [52].
2.1.6 Bone tissue engineering
Bone tissue engineering aim is to repair or replace lost large bone tissues caused by trauma or disease. By the use of scaffolds with the properties, porous, biocompatible, bioactive, biodegradable, high mechanical strength, sufficient to make it structurally stable for the formation of new tissue, and mimic the bone extracellular matrix (ECM), that provides the microenvironment for cell attachment, also, the use of bioactive agents promote cell migration, proliferation, differentiation, diffusion of oxygen and nutrients, this together produces new tissue formation and thus bone regeneration [56]-[58]. Bone scaffolds are better if their pores are distributed between 10-400 µm, with microscale and macroscale pores [56]. For cell infiltration the pore diameter is desirable about 200 μm [59]. These scaffolds cultured with osteoblast and stem cells showed biocompatibility, faster and deeper infiltration in scaffolds, cell viability, attachment and proliferation, osteogenic activities, biomineralization (calcium deposition), alkaline phosphatase activity (ALP), osteocalcin synthesis and enhanced compression modulus (Table 6) [56]-[62].
Choe et. al. 2020, fabricated a 3D scaffold by bioprinting and wet electrospinning using Core: polycaprolactone (PCL) (Mw: 80.000 g/mol) dissolved in methylene chloride (MC) /Shell: cellulose acetate (Mw: 30.000 g/mol) dissolved in dimethylformamide (DMF), collected in ethanol. The distance between the nozzle and the collector and the concentration of the solutions determined the effect of the coil structure and the homogeneity of the cellulose. 3D scaffold was evaluated in vitro using MG 63 cells showed osteogenic activities (ALP activity, calcium deposition, and gene expression) [59].
Alissa Alam et. al. 2020, produced polycaprolactone (PCL) (Mw: 70.000-90.000 Da)/ gelatin from porcine skin/ Poloxamer 188 scaffolds with Hexafluoro-2-propanolol (HFIP) as solvent, collected in water/ethanol. Ethanol-deposited scaffolds exhibited larger fiber diameter, with better mechanical properties and higher thickness compared to water-collected scaffolds. As future work the authors highlight the need to optimize coaxial wet electrospinning to produce scaffolds that can release both hydrophilic and hydrophobic bioactive agents with improved osteogenic properties. Scaffold presented controlled release the protein (Beta-lactoglobulin) and vitamin K2, additionally, enhanced Saos-2 cell viability and ALP activity [56].
Kara et. al. 2020, produced 3D nanofibrous fish scale/poly (3-hydroxybutyrate-co-3-hydroxyvalerate) (PHBV) (Mw: 80.000 Da) 3 % w/v, including an organic soluble salt BTEAC 0.2 % w/v dissolved in chloroform, collected in ethanol- water (9:1 v: v) as coagulant. The incorporation of fish scales into the PHBV scaffold increased biomineralization the deposition of Ca-P minerals, as well as the compressive modulus and higher bioactivity, MG-63 cell viability, alkaline phosphatase activity, and type I collagen production [57].
Dalgic et. al. 2019, produced 3D fibrous scaffold by co-electrospinning of poly (hydroxybutyrate-co-hydroxy valerate) (PHBV) / poly (ε-caprolactone) (Mw:80.000 Da) (PCL) 7/3 wt/wt at 14 % wt, with Diatom shells (DS), dissolved in Hexafluoro-2-propanol (HFIP), collected in ethanol as coagulant. In vitro studies showed that scaffolds bearing diatom shells (DS) a natural source for silicon, it was used as a doping agent that improved cell viability and proliferation of human osteosarcoma (Saos-2), additionally, showed osteocompatibility, alkaline phosphatase activity, and osteocalcin synthesis, osteogenic bioactivity [60].
Kim et. al. 2019, developed 3D scaffold of collagen type-I, extracted from porcine skin /PEO (Mw: ~900.000 g/mol) /PVA (Mw: 89.000 - 98.000 g/mol) in acetic acid as solvent, it was immersed in 1-ethyl-(3-3-dimethylaminopropyl) (EDC). The bone marrow-derived mesenchymal stem cells were cultured on the scaffold for 1 and 3 days, and exhibit cell viability and biocompatibility, the fibrous collagen structure provided a suitable microenvironmental condition to produce cell-substrate interactions [61].
Çatıker et. al. 2019, obtained porous 3D matrices via lyophilization and wet electrospinning of poly(3-hydroxybutyrate) (P3HB) (5 %, w/v) in chloroform as solvent, collected in ethanol as coagulant. Scaffold was reinforced with Poly-β-alanine (PβA), it demonstrated better cell attachment of MC3T3-E1 mouse pre-osteoblastic cell and proliferation compared to pure P3HB scaffolds, the addition of PBA had a positive impact on mechanical properties, such as elasticity, due to the decrease in crystallinity [62].
Colpankan Gunes et. al. 2019, prepared by wet electrospinning 3D scaffold of silk fibroin (0.5 % (w/v)) with poly (3-hydroxybutyrate-co-3-hydroxyvalerate) (PHBV) (Mw: 80.000 Da) (3 % (w/v)) and benzyl triethylammonium chloride (BTEAC) (0.2 % (w/v)) in chloroform as solvent, with hydroxyapatite (HAp) 0.3 % (w/v), ethanol-water (9:1 v: v) was used to collect the nanofibers. In vitro cell culture tests using MG-63 osteosarcoma human cells revealed improved biomineralization, cell viability, alkaline phosphatase (ALP) activity after 10 days of cell culture, the porous and interconnected nanofibers aided the growth and distribution of osteoblasts within the scaffolds [58].
2.1.7 Skeletal muscle tissue engineering
Skeletal muscle tissue engineering aims are to develop 3D substitutes for the treatment volumetric loss of muscle, alignment scaffolds produce signals that guide myotube, generating cell immersion and growing into the substrate [63], [64]. Additionally, for repair abdominal walls are commonly used synthetic polymers non-biodegradable as a mesh of polypropylene, polyester, and expanded polytetrafluoroethylene (ePTFE), in any case, the utilize of such materials can be related to complications such as seromas, fistulas, persistent quiet inconvenience, and surgical location infection [64], [65]. To solve this issue, biodegradable synthetic polymer scaffolds have been developed, which have a higher mechanical strength, allow tissue growth while avoiding infection, and adopt the physical properties of the abdomen [65], [66]. These scaffolds seeded with fibroblast, C2C12s cells and exhibit myogenic formation and proliferation, similar to skeletal muscle and was used as a replacement of the rat abdominal wall and showed an increase of vascularization, allowed mechanical stiffness and provided a healing at 8 weeks (Table 7) [32], [63], [65].
Table 7 Recent studies in wet electrospinning for skeletal muscle tissue engineering
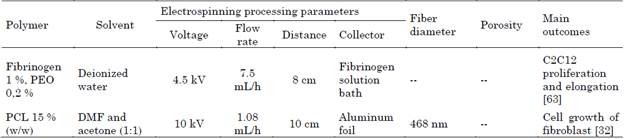
Source: Authors.
Guo et. al. 2019, generated 3D alignment scaffolds of fibrinogen 1 % /polyethylene oxide (PEO) 0.2 % dissolved in deionized water and collected in fibrinogen solution bath that contained Calcium chloride (CaCl2) and C2C12s myoblasts cells, these cells were immersed onto this scaffold, and exhibit myogenic differentiation and proliferation at 7 days of in vitro culture, conductivity, viscosity, surface tension and osmolarity of the solution help regulate the amount of electrical charge and mechanical shear to which the cells are subjected during the process [63].
Zhou et. al. 2019, created a 3D scaffold of PCL (Mw: 80.000 Da), 15 % (w/w) in dimethylformamide (DMF) and acetone (1:1) as solvent and collected aluminum foil, with aligned nanofibers and cultured with fibroblast per 7 days similar to skeletal muscle, the length-width and tilt angle of the double-bevel collector influenced the organization of the nanofibers within the 3D scaffold [32].
2.2 Industrial applications
Wet electrospinning for industrial applications has been used in water purification and air filter, biomedical sensors and flame retardants.
2.2.1 Water purification and air filters
Heavy metal ions in the water represent a health risk. Lead can be carcinogenic even at very low concentration, so it is desirable materials for the removal of heavy metal ions in water, with high ion adsorption. Nanofibers have the advantage of high surface area and the existence of abundant adsorption sites [67]. These nanofibers exhibit extraction capabilities for the removal of phenol, oil, chlorobenzenes from water (Table 8) [26], [67], [68].
Table 8 Recent studies in wet electrospinning for water purification and air filters
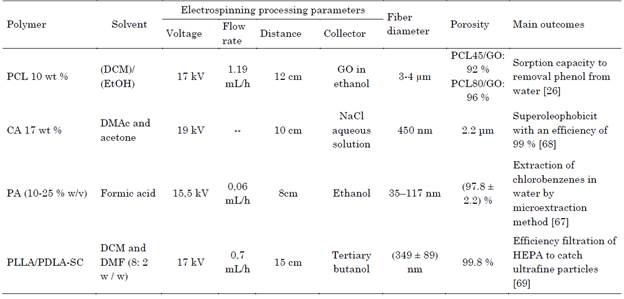
Source: Authors.
Maio et. al. 2020, development 3D scaffolds of polycaprolactone (PCL) (Mw: 45.000 Da and 80.000 Da), 10 % wt in dichloromethane (DCM)/ Ethanol (EtOH) (3:1 by volume) and collected in graphene oxide (GO) (0,5 mg/L) in ethanol, for phenol removal for water treatment, as a reusable green device that has sorption capacity, the surface tension of the ethanol allowed the polycaprolactone nanofibers to sink thus allowing the formation of 3D scaffolds [26].
Hong et. al. 2018, created 3D scaffolds of cellulose acetate (CA) (Mw: 70.000 Da) 17 % wt, in dimethylacetamide (DMAc) and acetone volume ratio of (2: 1), and collected in 150 mL of 1 M NaCl aqueous solution. 3D scaffold removed oil from water and showed excellent underwater superoleophobicity with an efficiency of 99 %, the remaining oil content was very low (<29 ppm), the membrane had a high oil/water separation flux and high oil intrusion pressure [68].
Bagheri et. al. 2018, fabricated 3D scaffolds of polyamide (nylon-6) (PA) (10-25 % w/v) in formic acid as solvent, and collected in ethanol. PA scaffold exhibit better extraction efficiency 8-10 times greater of chlorobenzenes (CB) in water than 2D nanofibrous, the limits of detection are in the range from 0.8-3 pg/ml. Results, verifies their suitability as an extractive phase in the method, needle trap microextraction (NTME) [67]. Because 3D scaffolds are more porous (up to 95 %), they allow a higher concentration of chlorobenzenes in water. Exposure to high concentrations has been shown to affect the brain, liver and kidneys, so the use of 3D nanofibers could provide a solution to this problem.
Current air filter aims are to eliminate ultrafine particles with a size smaller than 0.1 μm, to create a healthier environment. By the use of polymers with low cost and high performance to capture these particles, nanofibrous filters exhibit higher filtration efficiency than microfibrous filters, due to larger specific surface area that increases diffusion and intercept capture. Currently, high-efficiency particulate arrestance (HEPA) filters have a removal efficiency of 99.97 % for particles with sizes to 0.3 μm, but their efficiency for removal of ultrafine particles is relatively low [69]. For air filter have been developed nanofibrous aerogels of Poly(L-lactide) PLLA/ Poly(D-lactide) PDLA, exhibit good compressive resistance and combined with a layer of HEPA filter improve the filtration efficiency for ultrafine particles (Table 8) [69].
Ma et. al. 2019, designed nanofibrous aerogels of PLLA (Mw: 9.12 × 104 g/mol) /PDLA (Mw: 3.98 × 104 g/mol) 10 % wt dissolved in dichloromethane and N-N dimethylformamide in a ratio (8:2 w/w), and collected in Tertiary butanol. This scaffold exhibits good compressive resistance and combined with a layer of HEPA filter improve the filtration efficiency for ultrafine particles, attributed to the high porosity, as well as the slip flow effect of nanofibers [69].
2.2.2 Energy
Nanofibers in the energetic applications have been used due to their properties of large surface area to volume ratio that allow increased the energetic efficiency, high conductivity, flexibility and performance. The most important energy-related uses are as follows: Solar cells, Li-Batteries, Super-Capacitors, Fuel Cells, Nanogenerators, Hydrogen storage [3].
Although, multiple investigations with energetic applications have been carried out in electrospinning, the applications of 3D nanofibers produced in wet electrospinning have been little explored. Lithium ion batteries (LiB) store energy and have been commonly applied in mobile phones and laptops, due to their high energy density and long-life cycle [70].
Rechargeable lithium-O2 batteries have attracted more attention than lithium-ion batteries, due to their higher energy density (11.680 Wh/kg), they are more compact and lighter. Some problems with these types of batteries are limited discharge capacity and poor charge / discharge cycle stability due to a low pore volume utilization rate. Wet electrospinning allows the creation of a 3D network for manufacturing oxygen electrode materials, highly porous, with electrical conductivity and electrochemical activity, providing an interconnected oxygen diffusion pathway within the membrane, facilitating the oxygen reduction reaction (ORR) and the oxygen evolution reaction (OER) [3], [71], [72].
Lim et.al. 2019, prepared a nitrogen-doped hollow carbon fiber using polydopamine and electrospun polystyrene (PS) (Mw: 350.000 Da) 30 wt % in N,N-dimethylformamide (DMF), collected in ethanol as coagulant, and nitrogen-doped 3D scaffolds as sacrificial template, for the oxygen electrode of Li-O2 batteries. Due to the synergistic effects of nitrogen doping and hollow fibrous structure, the discharge capacity and recharge significantly improved, with an overpotential of ORR / OER reduced [72] (Table 9).
Supercapacitors (SC) or electrochemical capacitors are energy storage devices, applied in electricity and electronics. Due to its high-power output, long life cycle, fast charge and discharge times, and low maintenance cost. Depending on different energy storage mechanisms, SCs can be classified into pseudo-capacitors (PC) and electric double-layer capacitors (EDLC). PCs store energy based on rapidly reversible surface redox reactions (chemical adsorption / desorption), while EDLCs store energy by adsorption and desorption of ions at the electrode and electrolyte interface. Wet electrospinning allows the manufacture of flexible double-layer electrical capacitors (EDLC) based on highly porous nanofibers of polymers such as Polybenzimidazole (PBI) or polyaniline (PANI) with carbon or graphene nanofibers and metal oxides such as RuO2, MnO2 [3], [70], [71], [73]. The morphology and surface area of the nanofibers allow rapid access of the electrolyte to the electrode, resulting in higher energy storage density and rapid charge and discharge processes [73], higher than those of batteries [3].
Shi et. al. 2020, developed polyacrylonitrile (PAN) (Mw: 150.000 Da) 5 % wt, in dimethylformamide (DMF), and collected in a reduced graphene oxide (rGO) and ethanol. Double layer intercalated flexible supercapacitor (EDLC) electrode, for energy storage manufactured by a layer-by-layer wet electrospinning process with a dilute graphene oxide (GO) solution as coagulation liquid and subsequent thermal reduction treatment at 300ªC.
The rGO / PAN nanofibers demonstrated exceptional double-layer capacitance of 221 F / g at 10 mV / s, controllable electrical conductivity of 125 S / m, and stable cycling performance that retains a slight increase in capacitance after 10.000 cycles, NH3 gas released from the cyclization of PAN fibers induced N-doping on the rGO surfaces, further enhancing the charge storage capacity [74] (Table 9).
2.2.3 Biomedical sensors and flame retardant
Nanofibers with additives such as graphene oxide and carbon nanotubes (MWCNT), increases the electrical conductivity. Some polymers are also conductors between them polyacrylinitrile (PAN) and poly (3,4-ethylene dioxythiophene) poly (styrene sulfonate) (PEDOT: PSS), for applications as wearable strain sensors and conductive textiles. Silica nanoparticles (SNP) increase the flame retardant (Table 10) [74]-[77].
Shaker et. al. 2019, fabricated a sensible and conductive sensor of thermoplastic polyurethane (TPU) (Mw: 107.020 g/mol) at 10 wt % in dimethylformamide (DMF) and collected in poly (3,4-ethylene dioxythiophene) poly (styrene sulfonate) (PEDOT: PSS) 1.3 % wt in water and silicon rubber (SR) substrate, for sense rapid and minute deformation actions. The TPU/PEDOT: PSS strain gauge sensor manufactured presented a stabilized kΩ scale electrical resistance, regardless of the applied voltage and temperature value, demonstrated its durability and repeatability of use up to 15 cycles and its sensitivity to detect small loads [75].
Shaker et. al. 2017, fabricated nanofibers sensor of thermoplastic polyurethane (TPU) (107.020 g/mol) 10 % wt, in dimethylformamide (DMF) and collected in co-polymer poly (3,4-ethylenedioxythiophene)/ poly (styrenesulfonate) (PEDOT: PSS) 1.3 % wt in water and Kapton substrate, suitable for high temperature (90 ºC) strain sensing applications, the fabricated TPU/PEDOT: PSS scaffold showed a great improvement in electrical conductivity on the KΩ scale [76].
Nechyporchuk et. al. 2017, produced fibers from wood-derived cellulose nanofibers (CNF) (0.75 % wt) in deionized water and collected in 250 mL of aqueous solution of hydrochloric acid (HCl) at pH 2, and silica nanoparticles (SNP) at 44 % wt. The flame retardant until 600°C was attributed to silica nanoparticles that promote carbon formation and thermal insulation of the fiber surface was demonstrated, the incorporation of SNP on the surface of the nanofibers slightly reduced the toughness, however, preserved the Young's modulus [77].
3. PERSPECTIVES AND FUTURE TRENDS
Wet electrospinning is a technique that allows the development of 3D scaffolds with a high surface-to-volume ratio and an internal network of macropores, which improve cell infiltration for tissue engineering. Compared to traditional electrospinning, where packing density makes its pores nanometer-sized and hinders cell infiltration.
Wet electrospinning has several application areas, such as tissue engineering and industry. The parameters of the wet electrospinning solution and equipment influence the average diameter and morphology of fibers. For example, it is necessary to determine the right concentration of the solution to avoid fiber fusion and to maintain an adequate pore size to allow the formation of continuous nanofibers.
Environmental parameters (temperature and relative humidity) influence the reduction of nanofiber diameter and the increase of porosity, respectively. The equipment parameters (voltage, flow rate and distance between the needle tip) have influence on the morphology either in the form of ribbon or coil-fiber, the deposition time is associated with the thickness of the 3D scaffold, while, for the selection of the non-coagulant solvent used as collector influences the surface tension if it is very high (water: 72. 86 mN/m) the fibers float and if the surface tension is low (ethanol: 22.10 mN/M, chloroform: 26.67 mN/m, methanol: 22.50 mN/m, dimethylformamide: 36.40 mN/m) the fibers submerge, become more porous, less compact and with higher mechanical properties. The mixture of solvents in the ethanol/water coagulation bath with a surface tension lower than 28.52 mN/m allows continuous fibers to form, most works report a ratio (9:1) respectively. The use of surfactants reduces the surface tension of water, which improves fiber formation.
Long exposure to chemicals during the manufacturing process can be detrimental to cells; as a future prospect, tissue engineers should look to the use of non-toxic solvents in the coagulation bath.
Wet electrospinning allows the creation of microfibers, or nanofibers, from composite materials by combining natural and synthetic polymers. These scaffolds are chemically functionalized in situ in the coagulation bath to modify the surface of the fibers with additives such as nanoparticles, hydrogels, bioactive agents, proteins, drugs and growth factors, which increase mechanical properties (Young's modulus and compression) and improve cell attachment sites for tissue engineering and enhance properties for industrial applications.
Some research functionalizes 3D scaffolds with hydrogels to create an extracellular matrix (ECM)-like microenvironment, which has improved cell viability and proliferation.
It has been established that the required porosity percentage for tissue engineered scaffolds is greater than 80 %, which is significant for cell infiltration and necessary for homogeneous tissue regeneration. To date, in vitro studies with cells and some in vivo studies in animals have been performed. In vivo studies in humans using 3D scaffolds that respond to the specific needs of each patient are needed. Future work could explore the regeneration of other tissues, in which mesenchymal stem cell differentiation is stimulated along with bioactive agents.
Composite scaffolds improve their properties, more research is expected in the use of natural and synthetic additives (hydrophilic and hydrophobic) to provide better solutions to current treatments.
Research on conductive materials could lead to the development of new sensors, and research on carbon-based materials such as nanotubes and graphene oxide could contribute to the development of flame-retardant materials.
Wet electrospinning is expected to increase its industrial production of nonwoven fibers, for which the multi-needle technique has been used to increase productivity on a larger scale.
So far, wet electrospinning has demonstrated its applicability in interdisciplinary areas such as water purification and air filters, biomedical sensors and flame-retardant fibers, but other potential industrial uses need to be explored in future work.
4. CONCLUSIONS
Wet electrospinning is a technique of nanofibers scaffold fabrication that allows 3D structure, high porosity and high surface area. By a simple change in the metal collector of traditional electrospinning to a coagulation bath, many parameters have influenced in the fiber formation such as concentration of the solution, wet electrospinning configuration apparatus and the surface tension of the solvent. The fibers have micro porosity that improved cell infiltration, and with the addition of carbon nanotubes or graphene oxide increased the mechanical properties of the scaffold. Wet electrospinning can be development by coaxial technique to produce fibers with desired properties and structure core-shell and by multineedle technique to improve their production. Wet electrospinning has applications in tissue engineering and industrial applications. In the future is expected that other possible applications be explored thanks to the possibility of creating less packed and more porous fibers, which can be chemically functionalized in situ to obtain improved characteristics. The challenging aspects of wet electrospinning for future work are aimed at finding non-solvent solutions that are less toxic, but do not lose their effect on the formation of 3D nanofibers and specific design according to their application. In addition, a greater study of the physical and chemical properties of the polymers used in wet electrospinning is necessary in order to adapt their performance in terms of costs, biocompatibility and similarity with ECM for tissue engineering applications and improve the mechanical resistance for industrial applications.
One of the aspects to work on in the future involves 3D scaffolds morphology, and how these in the company of growth factors and bioactive agents influences the differentiation of stem cells. Finally, future work should evaluate the in vivo efficacy of 3D scaffolds for the regeneration of specific tissues, so far, few studies have evaluated its application in mice, but its use in humans could be extended with more in-depth studies.