Introduction
Lead (Pb) is a nonessential potentially toxic element (PTE) that naturally occurs at low concentrations in the environment (Adriano 1986; Alloway 1995). However, the excess of Pb is common in the soil due to anthropogenic activities. These activities are related to the use of this metal in industrial applications, mainly in the automotive industry, the improper disposal of mining and smelting wastes; air-borne sources; use of pesticides; biosolids and manure (Alloway 1995; Skerfving and Bergdahl 2007; Wuana and Okieimen 2011). Pb is also commonly found in urban landfill leachates as a result of the improper disposal of dangerous wastes. The contamination of the study region of this paper is associated with inadequate disposal of solid mining waste.
The contamination by Pb is a global concern due to its high toxicity and bioaccumulative properties. It can cause severe biological effects, increasing anemia, cancer and mortality rates (Lustberg and Silbergeld, 2002; Papanikolaou et al., 2005; Skerfving and Bergdahl 2007; Jin et al. 2008; Hegazy et al. 2010). The Joint FAO/WHO Expert Committee on Food Additives (JECFA) had established a provisional tolerable weekly intake (PTWI) of 25 Hg/kg by for all population groups. However, in 2010 the Committee concluded that this value could no longer be considered health-protective, and the PTWI of Pb was withdrawn (WHO 2011). There is no known safe blood level for Pb, concentrations as low as 5 ng/dL may be associated with decreased intelligence in children, behavioral difficulties and, learning problems (WHO 2018).
A recurrent problem related to Pb contamination is the inadequate disposal of solid mining waste. Pb is usually related to galena (lead ore - PbS) in mining areas, and during the extraction and beneficiation of ores, residues such as slags and tailings are generated. Their inadequate disposal can result in soil, water, and air contamination by Pb. The characterization of the soil is extremely important to assess the contamination (level and extension). It is equally important to have research efforts aimed at characterizing local soils to be used as liners in the waste disposal area. This may reduce costs involving the transportation of the wastes and/or materials for the construction of landfills.
In soils, the mobility and the fate of metals are regulated via their partitioning between soil and soil solution (Freeze and Cherry 1979; Lee et al. 1998). Metal mobility in the soil also depends on soil conditions and the chemical form of the metal (Alloway 1995; Rieuwerts 2007). Several studies have addressed metal behavior in different soils, such as Zuquette et al. (2008); Korf (2011); Braz et al. (2013); Odukoya et al. (2013); Diagboya et al. (2015), Zia et al. (2018); Gabarrón et al. (2018); Colzato et al. (2018). Other researchers specifically studied the use of tropical soils as liners for waste disposal (Boscov et al. 1999; Afolayan and Nwaiwu 2005; Knop et al. 2008; Boscov et al. 2011; Morandini and Leite 2015; Agbenyeku et al. 2016; Miguel et al. 2017; Onyelowe et al. 2019; Ehrlich et al. 2019).
Pb in the soil is cumulative and traditional treatments for metal contamination are very expensive (USDA 2000). Treatments can be done in situ or ex-situ, these include high-temperature treatments, washing processes and the use of solidifying agents (USDA 2000). For example, pump-and-treat is one of the most traditional techniques for remediation. But the high rate of energy required and the need for effluent disposal may increase the costs (USEPA, 2014). Thus, preventing metal contamination by using adequate liners is important to avoid the need for expensive remediation techniques.
Lateritic soils have been widely used in the construction of liners in landfill base (Boscov et al. 2011; Amadi and Eberemu 2012; Gabas et al. 2016). However, there is little practice in the use of cambisols as liners. There is increasing interest in these soils because of their availability in Brazil, or in other countries. According to Boscov (2011), it is important to expand the knowledge on the contaminant mobility behavior in tropical soils to encompass all the main types of soil of the country. Researchers have addressed this issue, such as Soares (2004), Morandini and Leite (2015), Almeida (2015), Colzato et al. (2018). Cambisols are very common, and the use of local soils in geoenvironmental applications may reduce costs, which is essential, especially in developing countries (Lavagnolo 2018; Shubbar et al. 2019). In this context, it is fundamental to perform a detailed study of the soil, covering its physical and chemical characteristics as well as its adsorption capacity.
The ability of soil to retain metals is directly proportional to its buffering capacity, and the retention mechanisms differ according to the pH values (Freeze and Cherry 1979; Bourg and Loch 1995). Depending on environmental conditions (pH, redox potential - Eh and temperature) and soil composition, metals can be retained in the form of oxides, hydroxides, carbonates, or confined in exchangeable cations and organic matter (OM) (Tessier et al. 1979). The OM content and the mineralogy of the soil can influence contaminant retention due to high cation exchange capacity (CEC) (Langmuir 1997).
Soil texture is also an important factor, as it can control the migration of contaminants through the soil profile. In soils with more clay content, soil texture favors the retention of metals. The retention capacity of PTE by adsorption is directly related to the surface area. Small particles in the clay fraction have higher adsorption capacity (Langmuir 1997) due to their large surface area.
Adsorption is the accumulation of a particular element or substance at an interface. The phase that adsorbs is referred to as the adsorbent and the phase that accumulates at its surface is the adsorbate. The nature of the force involved in the adsorption process may vary, they may be of physical nature, such as Van der Waals and electrostatic, or chemical, such as reactions with electron sharing (Weber 1972).
From the point of view of soil and groundwater contamination, the adsorption is the soil capacity to attenuate contaminant mobility and decrease the possibilities of these elements entering the food chain (Freeze and Cherry 1979; Sposito 1984; Sparks 1995). According to Alleoni et al. (2005), PTE soil adsorption depends mainly on: (a) clay content, (b) OM, (c) iron (Fe), aluminum (Al) and manganese (Mn) oxides content, (d) pH, (e) specific surface (SS) and (f) soil mineralogy. Braz et al. (2013) studied Pb, cadmium (Cd), cobalt (Co), copper (Cu), mercury (Hg) and zinc (Zn) adsorption by soils from the eastern Amazon, and the results showed correlation between the adsorption capacity and pH, organic carbon content and Fe and Al oxides content. Diagboya et al. (2015) also showed a significant role of soil organic matter and oxides content in adsorption of Pb, Cd, and Cu by four tropical soils from Nigeria.
The electrostatic charge of soil particles is linked to the adsorption rate (Zuquette et al. 2008). In tropical soils, these charges are highly variable (positive and negative) due to the mineralogical assembly and pH. For instance, Gupta and Bhattacharyya (2008) showed that the increase of pH led to increasing adsorption capacity of Pb by natural kaolinite. According to Mohamed et al. (2018), the pH effect in adsorption by soils is related to the changes in particle net proton charge. The electric charge of soil particles influences the adsorption of cations and anions, and the number of adsorbed ions depends on the charge density, related to the surface of the colloid, depending mainly on the type and content of mineral and organic constituents of soils (Alleoni et al. 2016). For a given pH, the net charge resulting from all sources of the surface of a colloid can be null, corresponding to the Point of Zero Charge (PZC). The Point of Zero Salt Effect (PZSE) is the pH value when the difference between the number of moles of H+ and OH- adsorbed is invariable with the salt concentration. If the charge densities of dissociated ions and adsorbed ions remain unchanged with the variation of the salt concentration, the PZC, and PZSE values are equal. However, this condition rarely occurs in soils (Sposito 1984; Sparks 1995).
The adsorption curve obtained in the batch equilibrium tests is useful to obtain preliminary information about the soil attenuation capacity (Daniel 1993). The adsorption isotherm is the ratio between the mass of solute adsorbed and the concentration at equilibrium. The isotherm describes the relation of the solute concentration between two separate phases in equilibrium at a constant temperature (Sparks 1995; Fagundes and Zuquette 2009). Thus, the sorption isotherm expresses the relation between the amount of vapor or solute adsorbed as a function of vapor or solute equilibrium concentration, not considering the mechanisms. The isotherm method consists of stirring soil subsamples with solutions containing increasing amounts of the metal ion. The amount of ion adsorbed by the soil is the difference between the initial ion concentration and the remaining ion concentration in solution subsequent to the experiment (Linhares et al. 2009).
Although the adsorption isotherm does not consider chemical mechanisms involved in adsorption, it allows describing the adsorption characteristics by the correlation between the parameters and soil properties (Vinhal-Freitas et al., 2010).
The study area is located in the Ribeira Valley region, which presents three major geological domains: pre-Cambrian metamorphic rocks (with oriented structures: schists, migmatite, and gneiss) and cataclastic (generated by shear stresses); magmatic rocks (represented by intrusive granite, basic and alkaline bodies) and Cenozoic sedimentary cover (CETEC, 1999).
The Ribeira Valley (Ribeira de Iguape water basin and Cananéia-Iguape estuarine complex) is a well-known region due to its historic Pb exploitation. The improper disposal of Pb slags in the soils and the upper Ribeira de Iguape river led to the contamination of many municipalities downstream, including the Cananéia-Iguape estuarine complex (Bosso and Enzweiler, 2002; Guimarães and Sígolo 2008; Rodrigues et al., 2012; Mahiques et al., 2013; Piedade et al., 2014; Kasemodel et al., 2016; Tramonte et al., 2016). There is a necessity of proper landfill implantation for the mining waste. Besides, landfills for urban wastes from the cities Itariri and Pedro de Toledo (both cities are located near Eldorado) were considered inadequate in the biennial report provided by the São Paulo State Environmental Company (CETESB, 2017). This problem entails the need to undertake studies that address the ability of local soils to adsorb Pb. The main objective of this research was (a) to determine the physical and chemical properties of a tropical soil (cambisol) collected in the city of Eldorado (Ribeira Valley), and (b) to evaluate the adsorption capacity of Pb by this soil.
Materials and Methods
Approximately 10 kg of soil was collected in the city of Eldorado (geographic coordinates: 24°31'12" S and 48°06'29" W), Ribeira Valley region, Brazil. The sample studied is a representative one, composed of the collection at 3 different points in the area. The disturbed samples, which were collected from B horizon, were air-dried at room temperature until constant weight, disintegrated with agate mortar and homogenized. Tests were performed in duplicates to determine the physical and chemical parameters of soil and the adsorption rates (batch equilibrium test) with Pb solution.
Particle size distribution
Special attention was given to the soil fine fraction given that the purpose of the study was evaluating Pb adsorption. A Micromeritics Sedigraph 5100 equipment carried out the particle size analysis, for particles up to 100 \im. The analysis was performed based on the ISO 13317-3/2001 method (ISO 2001). Soil (3.5 g) was mixed with distilled water to humidify with sodium hexametaphosphate - SHMP (1%, 50 mL) as a dispersant. The suspension was placed in Sedigraph and the sieved fractions were dried in an oven at 50 °C and weighed. The particle size and the granulometric fractions are estimated based on the Stokes law and the measurement was carried out by X-ray absorption (Lima and Luz, 2001). The determination was also carried out adding only distilled water to the sample.
Atterberg limits
The determination of the LL was performed according to ABNT MB30 (1984a) using the Casagrande apparatus. The determination of the plastic limit (PL) was performed according to ABNT MB31 (1984b). The plasticity index (PI), an important parameter used to evaluate the soil in the retention of contaminants, is the numerical difference between the LL and the LP (PI = LL - PL).
The activity index (AI) was calculated from the ratio between the PI and the clay content. This relation indicates the activity of clay in the soil. If the value of AI is less than 0.75, the soil is considered inactive; if the AI value is integrally comprised between 0.75 and 1.25, the soil is normally active and; if the AI value exceeds 1.25, the soil clay is considered active (Skempton 1953).
Physico-chemical parameters of the soil
The physical-chemical parameters determined were: (i) pH in water, in CaCl2 and in KCl solution; (ii) Eh; (iii) electrical conductivity (EC); (iv) point of zero salt effect (PZSE).
PH, Eh and EC
The pH was determined both in water and CaCl2 solutions (0.01 mol/L) based on the ISO 103090/2005 (ISO 2005) method. The relation between soil mass and volume of solution used was 1:5 (5 g of soil and 25 mL of water or CaCl2 solution). The suspensions were stirred for 5 minutes and then left resting for 24 hours. The measurement was conducted with a glass electrode and pH meter Hanna HI 9025.
The soil pH in KCl solution (1.0 mol/L) was obtained with a Digimed DH21 pH meter with a glass electrode. The relation between soil mass and volume of solution used was 1:2.5 (20 g of soil and 50 mL of KCl solution). The solution was stirred for 30 minutes and then left resting for 1 hour before the measurement (EMBRAPA 2011). The ∆pH is obtained from the numerical difference between the pH KCl and the pH H20.
The pH is an important parameter in the adsorption process. According to Fageria et al. (1994), the soil acidity is described quantitatively as slightly acid (pH between 6.0 and 7.0), moderately acidic (pH between 5.5 to 6.0), strongly acidic (pH between 5.0 and 5.5), very acid (pH 4.5 to 5.0) and extremely acidic (pH less than 4.5).
To determine the Eh value, a mixture of 1:2.5 (20 g of soil and 50 mL of distilled water) was prepared. The solution was stirred for 30 minutes and then left resting for 1 hour. The Eh was read using a platinum ring electrode connected to a pH meter Micronal B374, reference electrode Ag/ClAg.
To determine the EC value, a mixture of 1:2 (10 g of soil and 20 mL of water) was prepared and manually shaken for 30 seconds. The mixture was left resting for 1 hour before measurements were made using Hanna Instruments HI 9033 Conductivity Meter.
PZSE
PZSE is a pH value where the salt concentration of the solution in a soil suspension has no charge effect on the surface of soil particles. According to Silva et al. (1996) and Coringa and Weber (2008), the comparison between soil pH and PZSE allows to define whether the net surface charge of the particles is positive (pH < PZSE), negative (pH > PZSE) or zero (pH = PZSE). Soil PZSE depends on the content and degree of decomposition of organic matter and mineral composition of the clay fraction. To obtain the PZSE, a potentiometric acid-base titration was performed, varying to ionic forces. HCl was used as acid, NaOH as base and KCl as support electrolyte. In the first step, a series of solutions were prepared with increasing concentrations (0.002 M, 0.004 M, 0.008 M, and 0.016 M) of acid and base diluted in 0.1 M KCl, 0.01 M and 0.001 M solutions. Next, suspensions were prepared with 4.0 g of soil and 20 mL of each solution. The suspensions were maintained at room temperature for 24 hours with occasional manual shaking and pH values measured using a Digimed DH 21 pH meter. Equilibrium pH values (on the ordinate axis) versus the amount of adsorbed H+ (for the acid solutions) or OH- (for the basic solutions) were plotted. The PZC was obtained based on the equation proposed by Keng and Uehara (1973): PCZ = 2 pH KCl - pH H2O. The surface potential ( ) was obtained based on the equation proposed by Raij and Peech (1972):
= 59.1 (PZSE - pH H2O).
CEC and SS
The methylene blue method described by Arab et al. (2015) was used in this study. This test is based on the linear relationship between the methylene blue index and fundamental soil properties such as CEC and SS. Initially 2.0 g of soil sample was dispersed in 10 mL of water, under constant agitation with a magnetic stirrer. The suspension was titrated with a methylene blue solution (1.5 g/L). A volume of 1 mL of methylene blue was titrated in the soil suspension; the suspension was left mixing for 3 minutes. Then a droplet of the mixture was taken with a glass rod and placed on filter paper (Whatman n° 42). The test is considered negative if there is no presence of a light blue halo surrounding the droplet, and then the procedure is repeated adding an extra 1 mL of the methylene blue solution to the mixture. When a clear light blue halo is formed around the blue drop, the test is considered positive. According to Chen et al. (1974 apud Pejon 1992)1, the CEC is obtained by Eq. 1 where V is the volume of methylene blue (mL) used in the titration, C is the concentration of the methylene blue solution (normal) and M is the dry soil mass (g).
The SS values were empirically estimated using CEC results obtained by the Pejon (1992) method. According to Beaulieu (1979 apud Pejon, 1992)2 the specific surface is obtained using Eq. 2, where A is the area of the methylene blue molecule (130 Å), N is the Avogadro number (6.02 x 1023), m is the mass of dry methylene blue and MW is the molecular weight of methylene blue in anhydrous form. Eq. 4 is obtained by substituting the values.
A second method in accordance with ISO Standard 13536/1995 (ISO 1995), and adapted from Bascomb (1964), was used to estimate the CEC values. The method consists of saturating the soil with BaCl2 solution buffered to pH 8.1. Exchangeable cations in soils replaced by Ba were analyzed by atomic absorption. In order to precipitate barium adsorbed in the soil and the free cations in solution, MgSO4 solution was added to the soil sample. Magnesium occupies the exchange sites on the soil and the excess of Mg (not absorbed) is determined by titration with EDTA and Eriochrome Black T indicator.
The CEC is calculated according to the following formula:
where CEC is the cation exchange capacity in cmolc/kg; msoil is the weight in grams of soil used in the test; N is the volume in mL of EDTA used in the titration; M is the volume in mL of EDTA used in the blank titration; and B - A is the amount in grams of water in the soil sample.
The SS values of particles are related to the soil's ability to retain and/ or release soil chemical elements. Higher SS implies greater possibility of interaction with soil elements in solution, which can facilitate the retention process and attenuation of contaminants.
OM
OM content was obtained using the method described by Eusterhues et al. (2005). This method is conducted using a mixture 5 g of soil sample and 50 mL of hydrogen peroxide that is heated to 90°C. After the peroxide attack, the soil was oven dried at 60°C. The determination of the OM content is based on the mass difference of the sample before and after the procedure.
Pb concentrations
ICP-MS, analytical technique with high sensitivity was used to estimate Pb concentrations in soil samples. The analysis was performed after acid decompositions (HNO3, in Canada. HClO4, and HF) by ICP -MS at ACME Laboratory
X-ray diffraction (XRD)
XRD is a non-destructive technique used to identify the crystalline phases, which has been widely used in the identification of clays (Tan et al. 1986; Albers et al. 2002). For the total sample analysis, approximately 1g of soil was separated and gently broken down with the agate mortar. The sample was placed in an aluminum sample holder of the X-ray diffractometer, slightly compressing the powder to avoid a preferred crystal orientation. The diffractograms were obtained using the diffractometer Philips X'Pert consisting of a MPPC generator, PW3050 goniometer PW 3040/60 with a microprocessor in the printer registration. The radiation used was copper with a wavelength of 1.5405 Å. The diffraction angle 29 ranged from 4° to 65° at a rate of 0.02°/s. For the fraction analysis - diameter less than 0.063 mm - the clay thin-sections were prepared using a solution with about 1 g of soil sample, 30 mL of distilled water and two drops of sodium hexametaphosphate (1%) were added as dispersant. The mixture was stirred for about 40 seconds with ultrasonic stirrer. 1 mL of the solution was pipetted onto three glass slides; the droplet was left to dry at room temperature. One of the slides was subjected to treatment with ethylene glycol, another was heated to 500°C, and the third received no treatment. The three slides with different treatments were analyzed in the same diffractogram.
Differential thermal analysis (DTA) and thermogravimetric analysis (TGA)
This analysis was performed heating the samples to 1000°C at a rate of 12.5°C per minute. In the DTA a sample of the material to be studied was heated side by side with a sample of a thermally inert material (alumina) and the temperature difference between the two samples was recorded as the samples were progressively heated (Mackenzie 1957). The identification of minerals is by shape, position and intensity of the peaks of the curve produced in ATD. The obtained curve was compared with standard curves of various minerals (Santos 1989). In ATG, the sample weight was recorded as a function of temperature, while it was heated at a controlled rate. Changes in weight were assigned to rupture or formation of chemical or physical connections corresponding to the release of volatile substances or formation of compounds (Tan et al. 1986). The interpretation of the curves was made based on Mackenzie (1957) and Santos (1989).
Batch Equilibrium Tests
The study of adsorption is necessary to understand soil's ability to attenuate, degrade or immobilize contaminants of organic and/or inorganic origin. In the batch test method, soil and solutions containing the ion of interest are brought into contact, under agitation and during a predetermined period. At the end of the experiment, it is admissible that the system reached the equilibrium of the reaction (Soares and Casagrande, 2000).
In order to determine the sorption characteristics of the soil, batch tests were performed using solutions of five different PbCl2 concentrations: 20, 40, 60, 80, and 100 mg/L. This range was chosen based on Pb concentrations in leachate of mining tailings and slag from Adrianópolis (Ribeira Valley). These concentrations reached 77.10 mg/L (Raimondi 2014; Kasemodel 2017). For this test, we followed the methodology proposed by Roy et al. (1992) and Zuquette et al. (2008). The soil/solution ratio used was 1:5 (20 g of soil and 100 mL of solution). Samples were shaken in a NT 155 Novatecnica (between 25 to 30 rpm) benchtop orbital shaker. After 48 hours, the samples were centrifuged and filtered through Whatman filter paper n° 42. During the test, room temperature was controlled, and physicochemical parameters were measured. The pH, Eh and EC were measured before the test (solutions without soil sample), after soil and solution contact, and in the final stage, after equilibrium.
For the data interpretation the authors used the Linear, Freundlich and Langmuir isotherms. The linear isotherm corresponds to the simpler model of adsorption, and estimates the solute adsorption of infinitely increasing concentrations (Fagundes and Zuquette 2009). The linear isotherm model is represented by Eq. 6:
where: S is the mass of contaminant adsorbed per unit of soil mass (µg/g), C e is the final concentration of the equilibrium solution (mg/L), K d is the distribution coefficient of linear isotherm (cm3/g).
The Langmuir method, represented by the Eq. 7, uses two different linearization processes to obtain the parameters of this isotherm. The linearization shown in Eq. 8 and Eq. 9 are called Langmuir linearization I and Langmuir linearization II, respectively. In these equations: S m is the maximum amount of solute adsorbed by the soil (µg/g) and b is a constant related to the adsorption binding energy (cm3/g).
The Langmuir equation is often preferred since its parameters allow estimating the maximum adsorption capacity and adsorbate affinity of the adsorbate (Linhares et al. 2009).
The Freundlich isotherm is represented by Eq. 10 and linearization was used to obtain the parameters by Eq. 11. In these equations, S is the mass of contaminant adsorbed per unit of soil mass (µg/g), C is the final concentration of the equilibrium solution (mg/L), N (dimensionless) and K f (cm3/g) are coefficients of the Freundlich equation.
Results and Discussion
Physico-chemical and mineralogical properties
Table 1 presents the results of particle size distribution. As expected the experiment with and without sodium hexametaphosphate (SHMP) presented different results. For example, the clay fraction obtained in the soil when applying SHMP was 26.12% (Table 1), while the percentage of clay was lower (20.19%) without SMHP. Silt fraction was 52.47% with SHMP, and 63.09% without SHMP. The results of the test with dispersant show higher clay content and lower silt content. This is explained by flocculation of small clay particles when there was no dispersant.
Table 1 Particle size distribution of the studied soil in tests with and without dispersant.
Diameter | Distribution with SHMP | Distribution without SHMP |
---|---|---|
Hm | (%) | (%) |
0 - 2 (clay) | 26.12 | 20.19 |
2 - 60 (silt) | 52.47 | 63.09 |
60 - 100 | 0.79 | 1.18 |
> 100 | 20.62 | 15.54 |
According to Rowe et al. (1995), a clayey compacted soil can be used as a liner in a waste disposal area, if it presents 15 to 20% of clay fraction in its composition. According to the results (Table 1), this soil is suitable to be used in this application. Qian et al. (2002), indicates that a soil must to present at least 50% fines (silt and clay fractions) to be applied in a liner, a requirement that the analyzed residual soil also satisfies. The importance of fine particles in soils, used in the construction of liners, is to ensure lower hydraulic conductivity. Furthermore, particle size and sorption capacity are also related. According to Langmuir (1997), the sorptive abilities of minerals are proportional to their surface areas and surface-site densities. The bigger the proportion of fine particles, the larger the surface area exposed to reaction with the solution.
Table 2 summarizes the results of the physical, physico-chemical and mineralogical characteristics of the soil.
Table 2 Physical, physico-chemical and mineralogical characteristics of the soil.
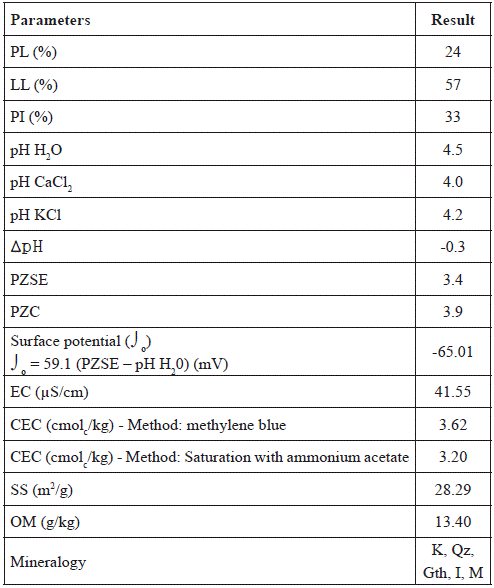
K: kaolinite; Qz: quartz; Gth: goethite, I: illite; M: muscovite
Soil LL was 57% and PL was 24%, therefore, the PI is 33%. This PI value is not within the ideal range for soil liners. Daniel (1993) recommends a minimum PI of 7% for soil liners to achieve the hydraulic conductivity required. However, the author also recommends special attention for soils with high PI.
According to Daniel (1993) and Rowe et al. (1995), soils with PI > 30%, such as the studied soil, may form hard clods that render difficult the construction of the liner, and may be susceptible to shrinkage and cracking if dry.
AI was 0.61 when using clay content without SHMP, and 0.79 when considering clay content with SHMP. Thus, the classification of the AI is dependent on the methodology used to obtain clay content, as when determining clay content without the use of the dispersant, AI is considered inactive; and when determining clay content with the use of SHMP, AI is considered normal. This is a positive aspect for liners because high activity clay soils are susceptible to shrinking and swelling, causing problems in the liner structure (Rowe et al. 1995).
The pH measured in water (pH 4.5) was higher than in CaCl2 and KCl solutions (4.0 and 4.2, respectively). In general and according to the results soil is very acidic. This may adversely affect the contaminant attenuation. Some metals, including Pb, remain soluble in acidic environments. In addition, pH influences the magnitude of the soil surface charge, which is also important for metal adsorption (Yong 2001).
The investigated soil PZSE (3.4) is lower than pH value in water (4.5), and ∆pH was negative (-0.3), indicating that the net surface charge of the particles is negative. The surface potential () obtained in this study was also negative (-63.24 mV). Similar values were obtained by Silva et al. (1996) in different soils collected from several regions of Brazil, and by Braz et al. (2013), in soils from eastern Amazon. The electric charge of soil particles influences the adsorption of cations and anions, and the quantity of ions adsorbed depends on the charge density, which depends on the colloid surface (Alleoni et al. 2016).
According to Sposito (1984), solid surfaces of soil particles and/or reactive minerals develop electric charge in two ways: (a) isomorphic substitutions between ions of different valences in minerals, or (b) from reactions of surface functional groups with ions present in the solution. These charges can be positive, null, or negative. According to Langmuir (1997), a sorbent phase endowed with a net negative surface charge exhibits a CEC, while a phase containing a net positive surface charge exhibits an anion exchange capacity.
CEC is a very important property that affects the retention of organic and inorganic species (Sposito 1984). The CEC of the total soil sample obtained using the methylene blue method was 3.62 cmolc/kg, while CEC obtained by NH4Ac saturation method was 3.20 cmol /kg. It should be noted that these CEC values are related to the total soil sample. If we consider that all that capacity is solely from the clay fraction (26% of the total soil), we can estimate the actual clay CEC dividing the total CEC by 0.26. This calculation results in the value of 13.8 cmolc/kg for the methylene blue method, and 17.9 cmolc/kg for the NH4Ac saturation method. In this context, we can estimate that the sample may have kaolinite, and a certain amount of illite as confirmed in the mineralogical analysis (Table 2). According to Langmuir (1997), CEC values related to kaolinite range from 3 to 15 cmolc/kg, which is strongly pH dependent, while for illite it ranges from 10 to 40 cmolc/kg, which is slightly pH dependent. According to Rieuwerts (2007) and Coringa and Weber (2008), well drained soils of humid tropical regions, such as those studied in this work, are intensely weathered and dominated by minerals with variable load, as previously proven, which is responsible for low CEC values, which could vary with pH.
The SS result of the residual soil was 28.29 m2/g. This result confirms the presence of kaolinite in the analyzed soil. Kaolinite SS ranges from 10 to 38 m2/g (Langmuir 1997). According to Sposito (1984), kaolinite SS ranges from 7 to 30 m2/g. The sorptive abilities of minerals are proportional to their surface areas and surface-site densities, which can range widely, reflecting differences in how they are measured (Langmuir, 1997).
The OM content of the residual soil was 13.40 g/kg, which is low. According to Rieuwerts (2007) tropical soils are relatively deficient in OM as a result of its rapid decomposition. But even these low concentrations of OM may be important for the soil CEC, mainly for soils dominated by 1:1 clays. Humic substances in OM can be highly reactive. OM can provide a higher CEC (150 to 300 cmolc/kg, according to Sparks (1995) in comparison with some minerals.
According to Alleoni et al. (2016), the presence of hematite, goethite and gibbsite explains the higher PZC values, and higher levels of OM and kaolinite are responsible for lower PZC values. The PZC value of the analyzed soil was 3.9, reflecting the presence of kaolinite. The mineralogical analysis indicated the presence of non-expansive clay minerals in the soil, such as kaolinite and illite (Table 2). According to Silva et al. (1996), the presence of kaolinite content in the soil explains the PZSE value. This mineral tends to contribute in lowering the average soil PZSE, due to its low isoelectric point (between 4.0 and 5.0).
According to Langmuir (1997), kaolinite is common in humid-climate, well-leached acid soils, and stream sediments where there is good drainage. Kaolinite results from weathering of the K-feldspar (microcline and orthoclase) and muscovite in rocks such as granite. Kaolinite is a low activity and non-expansive clay mineral (Mohamed et al. 2018). Illite clays may result from the weathering of micas and feldspars.
In addition to the non-expansive clay minerals, the analyzed soil is composed of goethite, quartz and muscovite. According to Rieuwerts (2007), the intense weathering and leaching observed in the tropics results in desilication, i.e. "the leaching of Si from silicates and the enrichment of primary quartz, secondary Al and Fe oxides, and two-layer clay minerals (mainly kaolinite) relative to all other soil constituents", as observed in the analyzed soil. According to Araujo et al. (2002), kaolinite and Fe and Al oxides are important components in the PTE retention.
Many authors studying the characteristics of tropical humid weathered soils reported the establishment of relations between the electrochemical, chemical and mineralogical attributes, which facilitates understanding the soil ion adsorption phenomena (Coringa and Weber 2008). In the batch test, it is essential to control the physicochemical parameters before and after the test. Thus, electrical conductivity (EC), redox potential (Eh) and pH measurements were measured before and after the batch test (Fig. 1).
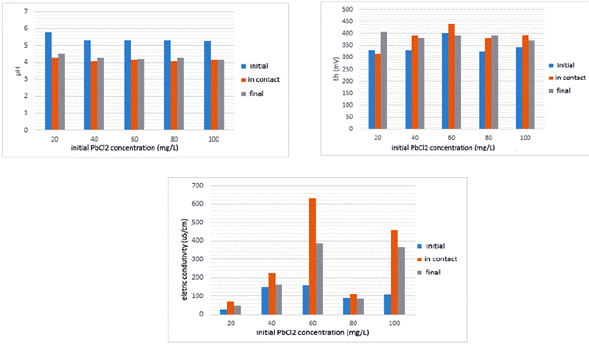
Figure 1 Values of pH, redox potential (Eh) and electrical conductivity (EC) of the solution used in the batch test, in the initial stage (t = 0 s) and final stage (t = 48 h) of the test.
The pH values of the soil in contact with contaminant solutions were within the range of 4.0 and 4.5. Results show little pH variation after the contact of PbCl2 solution with the residual soil. In this acidic environment Pb solubility is favored. The Eh of the samples ranged from 320 to 400 mV at the initial stage, from 310 to 440 mV at the contact, and from 370 to 405 mV at the final stage. These positive values show an oxidizing environment, which favors adsorption. Pb solubility is lower in oxidizing than in reducing environments. This is probably explained by the dissolution of Al, Fe and Mn oxides in reducing environments. These oxides play an important role in metal adsorption (Chuan et al. 1995). Significant variation of Eh during the test was observed for the samples with the solution of 20 mg/L and 60 mg/L PbCl2 After the contact between the 20 mg/L solution and the soil, the Eh increased 92 mV after 48 h. When using 60 mg/L PbCl2 solution, Eh decreased 49 mV after 48 h of contact with the residual soil. Other tested concentrations had minor Eh variations (Fig. 1).
EC decreased after 48 hours of soil solution contact for all tested concentrations, indicating a reduction in the concentration of salt solution due to the adsorption. A considerable EC decrease (approximately 40% of the initial EC value) occurs using 60 mg/L of PbCl2 solution, while smaller variations were observed using 80 and 100 mg/L of PbCl2 solution.
Batch tests
ICP/MS results show low concentrations of Cd (0.08 mg/kg), Pb (16.5 mg/kg) and Zn (19.4 mg/kg) in the studied residual soil. These concentrations are similar to results available in the literature (Wedepohl 1995) for soils collected in uncontaminated areas (0.10 mg/kg Cd; 17 mg/kg Pb, 52 mg/kg Zn). The results are also comparable to the background concentrations of PTE found by Nogueira et al. (2018) for natural soils from São Paulo state, Brazil (0.1 mg/ kg Cd; 10.1 mg/kg Pb, 21.8 mg/kg Zn).
Table 3 and Fig. 2 shows the percentage of PbCl2 adsorbed by the soil. The percentage of adsorbed PbCl2 decreased when the concentration entry was higher, which was also evidenced by EC measurements (Fig. 1).
Table 3 Data obtained during batch test: Pb initial concentration (C0 ); Pb concentration at the end of the experiment (C ); difference between the initial and equilibrium concentration (∆C); mass of Pb adsorbed by mass of soil (S).
Co (mg/L) | Ce (mg/L) | ∆C (mg/L) | S (µg/g) | Pb adsorbed (%) |
---|---|---|---|---|
20 | 1.50 | 18.50 | 92.17 | 92.5 |
40 | 3.82 | 36.18 | 181.35 | 90.5 |
60 | 7.74 | 52.26 | 260.78 | 87.1 |
80 | 12.20 | 67.80 | 339.34 | 84.8 |
100 | 18.26 | 81.74 | 407.88 | 81.7 |
As shown in Table 3, the adsorption capacity S of the soil increases with increasing solution concentration (C0). For the solution of 20 mg/L, soil adsorbed 92.17 µg/g. For the highest concentration (100 mg/L), S was 407.88 µg/g. It should be noted that the increase of the capacity S does not occur at the same rate as the increase in concentration, i. e. the isotherm is not perfectly linear. Such behavior is typical of dissolved species in soils. At low Pb concentrations, the affinity of the metal with the solid surface of the soil is higher. At increasing concentrations, as the adsorption sites are occupied, this affinity decreases. Consequently, the highest percentages of Pb adsorption were obtained when the lowest initial concentrations were used (Langmuir 1997). Based on Table 3, using the initial concentration 20 mg/L, the percentage of lead adsorbed by soil was 92.5%. For the solution with 100 mg/L of Pb, the adsorption percentage is comparatively smaller (81.7%).
The Figure 3 presents the Langmuir I and II, Freundlich and linear isotherms. Table 4 shows the data obtained when using Langmuir I and II, Freundlich and linear isotherms.
Based on the correlation coefficients obtained from Linear, Langmuir and Freundlich equations, all models adequately describe the experimental Pb adsorption results. The Langmuir II (R2 = 0.9982) and Freundlich (R2 = 0.9927) models had the best adjustments (Table 4). The Linear equation had the lowest adjustment compared to Freundlich and Langmuir I and II. Similar results were obtained by Linhares et al. (2009) studying the Pb adsorption in highly weathered tropical soils collected in the region of Minas Gerais (Brazil). For these authors the adsorption was best represented by Langmuir (0.98 < R2 < 0.99) and Freundlich (0.94 < R2 < 0.99) equations. Freundlich equations had also provided better adjustments for Pb adsorption by 4 soils from Goiás state (0.96 < R2 < 0.99) (Oliveira et al., 2010) and by a transported soil from Ribeira Valley (R2 = 0,98) studied by Araujo (2015). Paranavithana et al. (2016), observed that the Langmiur isotherm presented the best fit for Pb2+ and Cd2+ in coconut shell biochar (fine granules), in a local soil from Sri Lanka, and in a biochar-mixed soil (1:1 mixture of biochar and soil).
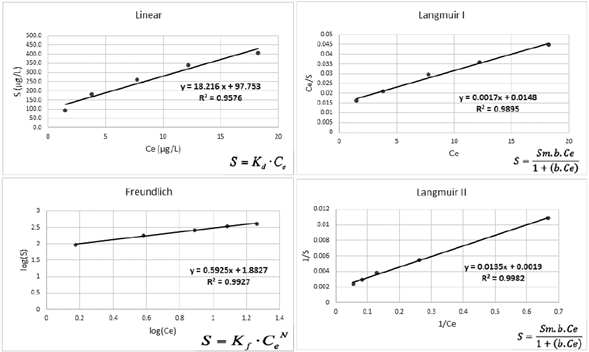
Figure 3 Linearization graphs of the linear, Freundlich and Langmuir (I and II) adsorption isotherms.
Table 4 Adsorption isotherms parameters: distribution coefficient of Linear isotherm (Kd) Langmuir maximum adsorption capacity (Smax); Langmuir adsorption constant related to binding energy (b); Freundlich equilibrium constant (Kf); Freundlich exponential coefficient (N); correlation coefficient of the isotherms (R2).

The parameter b (Langmuir), which is related with the adsorption energy, was 0.1149 cm3/g for Langmuir I and 0.1407 cm3/g for Langmuir II (Table 4) models. According to Linhares et al. (2009) the Pb is an easy adsorption cation. According to Pierangeli et al. (2001), this element is retained initially by negative charges of the surfaces of soil colloids, later forming more stable bonds. In the latter case, according to these authors, Pb becomes part of the surface of colloids and available to plants in limited quantities. The Pb interactions with soil colloids are predominantly more specific and less dependent on surface charges. The specific adsorption involves the exchange of metals with ligands present on the surface of the colloids, which forms covalent bonds and is assumed as the cause for which some soils adsorb metals above their predicted capacity based on CEC (Linhares et al. 2009). In soils with variable loads, it is difficult to distinguish the contribution of each of its components in the adsorption of metallic cations. Even when regarding pure minerals, the adsorption capacity of a certain oxide or clay mineral depends on its crystallinity degree, which is extremely variable with the age and environmental conditions of its formation (Pierangeli et al. 2001).
The maximum adsorption capacity of the soil is 588.2353 µg/g. This value is lower than the capacity of transported soils from the same region. Rodriguez (2013) and Araujo (2015) studied Pb adsorption by 3 transported soils from Ribeira Valley, using batch equilibrium tests (with soil-solution ratio 1/5). The authors reported maximum adsorption capacities varying between 893.00 and 1111.11 mg/L.
Even so, the soil studied in this research proved to be efficient to adsorb Pb in solutions with concentrations close to those found in leachate from mining waste. Kasemodel (2017) studied soil and slag samples from a waste disposal area in Adrianópolis. The Pb concentrations found in the leach test extracts ranged from 5.16 to 77.1 mg/L. Raimondi (2014) studied mining tailings also from Adrianopolis and the leach extracts showed Pb concentrations varying from 13.5 to 77.1 mg/L. For this range of concentrations in batch tests, the soil presented adsorption efficiency higher than 84.8% (for the C0 = 80 mg/L).
Conclusions
The tropical soil (cambisol) presented some favorable physical and chemical properties, and others less suitable, to be used in the construction of liner in the waste disposal area. The soil is fine and its granulometry is compatible with that recommended in the literature for use in liners of compacted clay. The clay fraction is composed of 1:1 clay minerals such as low activity, non-expansive kaolinite. The oxidizing medium and the predominance of negative charges on the surface of the colloidal particles (evidenced by delta ∆pH = - 0.3 and PZSE = 3.4) favor the retention of metallic cations.
On the other hand, some characteristics of this tropical soil are less favorable, when observed separately, for the retention of contaminants. This is the case of low CEC, which may result from the presence of kaolinite. Low OM content and acidic pH also discourage contaminant retention. In addition, the PI of 33% is in the limit of the recommended range, thus, the material can be used as a liner.
As for adsorption, it was noted that the highest percentages of Pb adsorption were obtained at the lowest initial concentrations. Thus, the adsorption percentage of Pb when using initial concentration of 20 mg/L was 92.5% and, when the initial concentration was 40 mg/L adsorption rate was 90.5%. For higher Pb concentration (100 mg/L) the adsorption percentage is comparatively smaller (81.7%). Based on the correlation the Langmuir II (R2 = 0.9982) and Freundlich (R2 = 0.9927) models had the best adjustments. The results indicate that the maximum adsorption Smax (Langmuir) was 588.23 µg/g for Langmuir I and 526.31 µg/g for Langmuir II models. Thus, it is concluded that the tropical residual soil presents characteristics that allows it to be used as liner in the disposal of residues containing Pb. But we recommend the study of possible additives to the soil to improve the plasticity and ion exchange characteristics.